Flash and JavaScript are required for this feature.
Download the video from iTunes U or the Internet Archive.
Topics covered: Magnetism and spectrochemical theory
Instructor: Catherine Drennan, Elizabeth Vogel Taylor
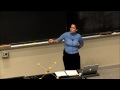
Lecture 30: Magnetism and S...
Related Resources
Lecture Notes (PDF)
The following content is provided under a Creative Commons license. Your support will help MIT OpenCourseWare continue to offer high quality educational resources for free. To make a donation or view additional materials from hundreds of MIT courses, visit MIT OpenCourseWare at ocw.mit.edu.
PROFESSOR: OK, everyone, pay attention to the clicker question. If you haven't responded, now's a good time to click in your response. All right, let's just take 10 more seconds. OK, we can do better than this.
So, tetrahedral complexes. Do you recall tetrahedral complexes with angles of what between the ligands? 109 . 5. The ligands' negative point charges aren't facing any of the d orbitals perfectly. They're a little bit closer to the orbitals that are 45 degrees off-axis, so those three are the most repelled. But they're not really directly hitting any of them. So that's in contrast with the octahedral system or square planar where the ligands negative point charges are headed directly toward some of the d orbitals. So because the ligands in a tetrahedral case are not headed directly toward any of the d orbitals, there is not a huge amount of crystal fields splitting, so that's small.
And so, when the splitting is small, then you tend to have high spin systems. So you put in all of the electrons singly to the fullest extent of the orbital as possible before you pair. And so, since you put them in singly for the fullest extent possible before you pair them up, that will lead to a high spin system, which is the maximum number of unpaired electrons.
So today is then the last lecture on transition metals, and we've been talking about crystal field theory, and today we're going to talk about colors and crystal field theory. So, colors, there are a lot of beautiful colors in nature, and some of the beautiful colors you find in nature have to do with transition metals or other liganded states. So, we're going to start with an example of how colors can change, how a molecule's color can depend on its oxidation state, how a molecule's color can depend on its liganded state. So, Dr. Taylor is going to be doing this for you -- should we do it the demo and then look at the questions or do the questions first? Demo first?
PROFESSOR: OK.
PROFESSOR: So, here are some of the reactions up on the Powerpoint that you're going to be looking at, and as the reaction proceeds, there's going to be changes in oxidation state, and also changes in ligand in state, and that will lead to changes in color.
[DEMONSTRATION]
PROFESSOR: So, how would you describe that first color? Any predictions? Have any of you seen this before, what's going to happen next?
[DEMONSTRATION]
PROFESSOR: So this is called an oscillating clock reaction, so as it runs through, it cycles between the different colors. So, that should happily keep going, and we can consider what's happening. So here's the overall reaction here and it can be divided into two components. So, why don't you tell me, what is happening to iodide in that first reaction, and there it is again. All right, so let's take 10 more seconds. Very good.
So, if you looked up here, you see that you have minus 2 for the oxygen, three of them, minus 6, and it needs to equal minus 1, so you have plus 5. And then over here, we again have plus 1 minus 2, and so that has to be plus 1. So that was actually a quiz question, but you score three points if you answered, even if you got it wrong, but we could have had that four points, most people got that right anyway. But if you answered, you get an extra few points on that one.
All right, so very good. That's what's happening to iodide. Now you notice that in this reaction h o i is being produced, and then in the second step it's being consumed. This later reaction can be divided, then, into two additional reactions. And one of the reactions, you can tell me again what is happening, what is being oxidized and what is being reduced in this part of the reaction. OK, let's take 10 more seconds. Excellent. Even a little higher than before. All right, so you figured out that this was the plus 1, this is minus 1, and they're both going to 0. So you have oxidation and reduction going on all involving iodide in that reaction.
OK, so as the reaction was proceeding, you could see that it started out with clear and then it went to sort of amber color, and that was the i 2, the clear was i minus, and the sort of darker blue color that you saw is the complex with starch in the reaction. So that both of these guys have colors independent, but then when they're liganded to something else, the color is different. And that's very true with coordination complexes that the metal by itself will be strongly influenced by what the ligands are. And depending on what type of ligands it has, it can have a really entirely different color than it had before.
So, today we're going to talk about why that's true, and how you can predict colors based on what type of ligand is bound to a transition metal.
So, a lot of transition metals have really beautiful colors, and my laboratory studies metals bound to proteins, and often the proteins will have really beautiful colors because of the metal cofactor involved, and that's one of the things that I liked about that particular area of study is just how beautiful these proteins can be. So, the color given off depends on the nature of the metal, and depends on the nature of the ligand. And so we can use crystal field theory, which again, is a very simplified theory, to try to predict or explain the observed colors. And so again, this is not always very precise, but given, if you're told information out of color, you can rationalize why that would be true, and you can also predict at least a range of color that you would have under certain circumstances.
So, let's take a look at this more. So the ligands again, have the ability to split those d orbitals. And when we're talking about for metals, it's all about the d orbitals. And we talked already about strong field ligands and weak field ligands, and we're going to talk more about that today, but this time in terms of color. So a strong field ligand, as we discussed, creates a big splitting in the d orbital energy, whereas weak field ligand, like the first question you had today with tetrahedral complexes, there's usually a weak field there, so we have a small energy separation between the d orbitals.
So here is something that you actually have to memorize -- there's not much memorization in this course, but you do need to memorize these six ligands in terms of their ability to split d orbitals. So, on the side, we have three that are strong field ligands, cyanide, c o, and ammonia, and so those are going to be strong field, so they're going to have big splitting energy, and so they'll tend to be low spin. Then we have three that are sort of in between that are intermediate field -- water, hydroxide and f minus.
And so, in comparing, those are intermediate, so you're going to be asked questions such as how does that compare to a weak field, how does that compare to a strong field. And then our weak field ligands or a lot of our halides down here, i minus, b r minus, c l minus. And so those are weak field ligands, so you'll have a small splitting, so they'll tend to be in high spin complexes.
So let's take a look at some examples. So we talked about iron before in complexes, and now we can consider two cases where we have iron plus 3, so the same metal in the same oxidation state, but it has different ligands. It So in one case you have a high spin system with six water ligands, and then the other in a low spin system with six cyanide ligands. So first, before you do anything else with this, you always have to think about what the d count is. So, to do the d count, we're going to look at where iron is in the periodic table, and we're going to see it's in group 8. And then, we'll have 8 minus 3, the oxidation number is d 5 system.
So now we have two diagrams here, one has a big splitting, one has a small splitting, and why don't you fill in for me in a clicker question, what the high spin system would look like. OK, let's just take 10 more seconds. Very good. I think that's one of our highest numbers in a while.
That's right, so we're going to fill to the fullest extent possible before we pair any of the electrons. So again, here we put in electrons down here, and then go up here, because the splitting is small, so it doesn't take that much energy to put an electron in the upper orbitals, it takes more energy to pair the electrons for this weak field system. And so, this is a high spin case, we have a maximum number of unpaired electrons.
So, over here when we have a much bigger splitting energy, it's going to take a lot more energy to put electrons up there, and so we're going to fill up all of these orbitals down here until we have to put an electron up there. So, if we do that, put in the three, and now we're going to pair, because it takes less energy to pair than it does to put an electron up there, so we do four and five. And so then here is our system where we have a strong field, and so here's a weak field and it's going to be high spin, maximum number of unpaired electrons, here we have the strong field and it'll be low spin, the minimum number of unpaired electrons. And we're doing this, again, because we know that cyanide is a strong field ligand, whereas water is an intermediate field ligand, it's a lot weaker than cyanide.
OK, now we can continue doing some of the things that we've done before. So just to review this material, we can write the d n electron configurations. What are the orbitals called that are down here? Yup, t 2 g. And how many electrons do we have there? three. And then the e g system up here with two electrons. And over here what do we have? Yup, t 2 g to the 5. So, a review, electron configurations, these are just shorthand notations, which tell people what these diagrams look like.
Then, we also talked about this before. So, what does this term stand for? Crystal field stabilization energy, right. So now why don't you tell me what it is for the high spin system. OK, let's just take 10 more seconds. Yup, zero. So if we look at that, we have three electrons down here, so that's minus 2/5, two electrons up here, which is plus 3/5, so 3 times minus 2/5 is minus 6/5, plus 6/5 gives you 0. So here is a case where you really don't have much stabilization. It would be equivalent then, so there's zero stabilization because you have three electrons down and two up.
All right, so what about the low spin system now? So if we can look at that, what are we going to have for this system? So, we have 5 times minus 2/5 or minus 10/5, and we also have two pairing energy terms there, which we can write in, because there are two sets that are paired.
So this is mostly review on what we've had before, and we haven't talked about some of these things in a little while, so we go over it again, and now we're going to take it a next step and think about what sort of wavelength would be absorbed if you're going to promote some of these electrons to unfilled orbitals. So what about the light absorbed by these octahedral coordination complexes? So, if you remember back to the beginning of the course, a physics course or high school, a substance absorbs photons of light if the energy of the photons match the energy required to excite those electrons to a higher energy level. And so now we are doing some review from the first part of the course, which is always good.
As I mentioned, everything kind of comes together and we need to go over everything for the final, but there's also connections between all the different parts of the semester. So this should look familiar to you. So, the energy of the absorbed light equals Planck's constant times the frequency of that light. But now we can make that equal to another term, a term we've been talking about in this unit, and that is our octahedral crystal field splitting energy. Because the energy that's going to be required to bump an electron from here to here is that energy, that splitting energy. So that's going to be equal to this term here.
OK, so what does this mean in terms of the wavelengths of lights absorbed by different coordination complexes. So we can think about that. So if you have high frequency of light is absorbed, the wavelength of the absorbed light is going to be short. And we know that relationship -- again, think back to the beginning of the course and also probably to physics and to high school, we know a very handy equation for telling us about the relationship of frequency and wavelength of light, so we have the speed of light equals the wavelength times the frequency. So if you have a high frequency of light absorbed, the absorbed wavelength is going to be short.
So, let's look at a couple of examples, the example here, going back to our example. So we had this high spin system with water, and now I'm telling you that the splitting energy is 171 kilojoules per mole, and when you have cyanide as your ligand, your splitting energy is 392 kilojoules per mole. Again, this was a stronger field ligand, so we have a bigger splitting energy. And this was an intermediate field ligand, certainly weaker than cyanide, so this has a smaller splitting energy.
So, from these values now, we can calculate the wavelength of absorbed light. So for the high spin system first, we can rearrange these equations, which you know well, to come up with the rearranged equation, the wavelength equals Planck's constant times the speed of light, and divided by e, and this time our e is that crystal field splitting energy. So we can put in these terms. So we have Planck's constant times the speed of light over our octahedral field splitting energy, oh, but then we have some other terms here. Now one thing that you have to pay attention to in this unit is your units. So, splitting energies are often given in kilojoules per mole, whereas you often see Planck's constant in joules, so we want to make sure that we convert one or the other, and here it's set up to convert the kilojoules to joules. And also, we want our final unit, we're talking about wavelengths, in meters or nanometers, so we need to get rid of this mole term, and we use Avagadro's number to do that.
So now we should be able to cancel our units and get the correct units. So we should be able to cancel the seconds over here, and we should also be able to go in and cancel our moles. We should be able to cancel the joules and the kilojoules out, and that should leave us just with this over here, which is meters. So this is 7 . 0 0 times 10 to the minus 7 meters. Does that make sense in terms of a wavelength of light? Because that would convert to what nanometers? 700 nanometers. So if you do something strange and you forget Avagadro's number, you're going to come up with a very interesting wavelength. So that's a good way to check to make sure that you've done the problem correctly. So, 700 nanometers, anyone remember what light that is, what color that corresponds to? Red, so it's absorbing red light.
All right, so now let's just do the same thing for the low spin system with the cyanide ligand, and we're going to plug in our 392 here, and we get 305 over here. And so, that is a much shorter wavelength of light. So again, light absorbed for the compound with water, 700 nanometers, and the compound of iron with cyanide, 305, and so we're absorbing a red light over with the water compound, and sort of purple or violet light is being absorbed in the cyanide complex. And we're talk in a minute about the light that is being transmitted, which is complementary to the color of the light absorbed. So by knowing something about splitting energies, by knowing something about the types of ligands, then you can know something about colors.
So now, for another example, we're going to look at the different colors of two chromium complexes. So first, what is the oxidation number of chromium in the water complex here? What is it? And what about over here with n h 3 ligands. Plus 3. So, plus 3 and plus 3. And what is our d count? You know where chromium is, in what group? Six. 6 minus 3 is 3, so we have a d 3 system. What does c n mean again? Coordination number, so what is that for both of these? Six. So there's Six things coordinated to the chromium, and so we have, again, an octahedral system. And what type of ligand is water? Intermediate. And what about n h 3? Strong. So this is strong, and water is and intermediate ligand, and certainly, it is weaker than n h 3. So, we expect one system over here for a strong ligand, and then something that's weaker than that strong ligand.
All right, so here are two diagrams, one with a big splitting energy, and one with a smaller splitting energy. Are the diagrams going to look same or different? The same. Because we only have three electrons, so they're going to be the same. In both cases, we put in the three electrons in the lowest orbitals, and then there's no decision to be made, because there isn't that fourth electron, so we don't have to decide which place to put this.
So these diagrams are going to look the same, and before when we were doing this in this unit, we said OK, we're done, they look the same. But now, we realize that these are really not the same compounds and that they're going to have different properties, even though their diagrams are going to look the same. Because the energy that it's going to take to excite an electron here is much smaller than over here, and that's going to result in a different wavelength of light being absorbed in these two different cases, which will mean a different wavelength of light being transmitted. So, again, here we have a weaker field and here we have a stronger field.
So again, we can go through and think about these two cases. So when we have a smaller term here, that means a lower energy -- this is a splitting energy, and so we'll have a lower frequency. When we have a larger case or higher energy, we have a higher frequency. Again, if you have a lower frequency absorbed, we have a longer wavelength absorbed, and in this case, the higher frequency translates into a shorter wavelength absorbed.
The color of the transmitted light is complementary to the color of the absorbed light. So now we can think about -- I always want to ask, when do people learn about complementary colors? Is that 6th grade, earlier? I don't really remember, but I think it's pretty early on. And people always ask me, are you going to have that on the equation sheet, or do I have to actually remember my complementary colors? And I tell people that I will put some version of this on, so you don't have to review your kindergarten notes for this class, if that's when you learned it. It's pretty early, I don't know when it is exactly.
So, the color of the light is going to be complementary about -- this is very approximate. So here, if the transmitted light is shorter because we have this weaker splitting, then we are expecting we're going to have this shorter wavelength, the transmitted light, and experimentally, if you make this compound you'll see it's violet. In this other case, we have a stronger field ligand, and so you have a larger energy, higher frequency absorbed, shorter wavelength absorbed, then you're going to have a longer wavelength from your transmitted light. And that this compound, if you make it, is actually yellow.
So if we go back to our colors for a minute, you see that when you have the shorter wavelength, we have a violet, and that is a short wavelength. And in this case for the strong field ligand, we are going to have transmitted light of a longer wavelength and it's yellow. So it's the same oxidation state of chromium, it's the same -- it's an octahedral complex, six ligands in both cases, same octahedral crystal field diagrams, but yet one compound has a violet color, and the other one has a yellow color.
All right, so one can also be asked to calculate a crystal field splitting energy in kilojoules per mole, given the appropriate information. So we've looked at when a splitting energy is given, and we've been asked to calculate wavelength absorbed, you can also be asked to go in the other direction. And so here we have another chromium complex to work with. And we're told that the wavelength of the most intensely absorbed light is 740, and so what would you predict the color of this to be? It would be greenish. So that would be what you would predict. Again, chemistry is an experimental science, but based on having a complementary color to the one absorbed, that would be a guess.
All right, so we can actually calculate the frequency of the light absorbed. So we were given the wavelength, and use speed of light, and plug in your wavelength and you can come up with a frequency, 4.05 times 10 to the 14 per second. Then we can calculate from that the crystal field splitting energy, and so we use Planck's constant, and we have our frequency, and we calculate 2 . 6 8 times 10 to the minus 19 joules. Am I done with the problem? What does the problem ask for? It asks for it in kilojoules per mole, and so, we're not done, we need to convert to kilojoules per mole. And I'm making this point, because often this is where people lose points on the final exam, and that's not where you want to lose points. You want to lose them on a really hard problem, not on something like this.
So, most of the time you're asked for kilojoules per mole, so make sure that if that's what asked for, that's what you provide. So here, we can just do the conversion of units, and then we're going to use Avagadro's number to give us that per mole. And so, this translates into a 160 kilojoules per mole, which you might recognize is more similar to the other numbers that you saw for octahedral crystal field splitting energy.
All right. Sadly, there are some coordination complexes that do not have colors. Why would that be? Why would something not have a color? It has d orbitals, it's a transition metal. So what would be true about all of the d orbitals? Yeah, so one example, is if they're all full, and that is the most common thing that we see, so it's not possible to have a d to d transition in the visible range.
So there are a number of examples of metals who have this situation. Zinc and cadmium are two of the most common that give you problems in biological systems. So why is this? Well, that's because they're over here in group twelve. But their most common oxidation states are plus 2. So, if you have 12 minus 2 you have a d 10 system, and that's the case for both of these systems here. And so all the d orbitals are filled.
Now, zinc is a really important metal in biological systems, and because it has all these d orbitals filled, it doesn't have a color. And so it's very hard to tell if an enzyme molecule has zinc. And I think one of the problems that you have on this problem-set talks about how zinc is important in a biological system by altering the p k a of a residue that coordinates to it. And that's often its job, and so biochemists are often faced with the problem of trying to figure out if their protein has zinc, but they have no color of the protein, also they might try to look for a paramagnetic or diamagnetic system. They're not -- you know if they see a paramagnetic system, they say oh, unpaired electrons, we know we must have metal involved, but there's no sort of spectroscopic probe for zinc.
And so, often someone will determine a crystal structure, and it'll be a huge surprise that there's zinc associated with this protein. Do you think there's a lot of proteins that use cadmium as of part of their mechanism? What do you know about cadmium? Yeah, cadmium is poisonous. Old barbecue grills were sometimes, they used to coat things with cadmium on a barbecue grill. Yeah, that was not very smart. So, cadmium poisoning is a problem, and people have been trying to figure out the mechanism of that, but again, it's hard to study cadmium because it has no spectroscopic signal.
All right, so getting back now to just kind of review over what we've talked about in terms of colors. So we have our weak field ligands, again, you need to memorize what they are. You have your intermediate field ligands, which you need to memorize, and also your strong field ligands. So these weak field ligands are going to have a small splitting energy, and that means that in terms of how the complex absorbs, low energy, low frequency, long wavelength, and that the color transmitted will be complementary. And usually what this means is that it'll be sort of in the end of the spectra, so it's often hard to say well, red will definitely be green. So it's not a perfect agreement, but you can usually say well, it's probably going to be in the blue-violet or green end. So in sort of one part of the spectra.
Strong field ligands, again, have a huge splitting energy. So you're going to have big energy, high frequency, short wavelength, and so it's going to transmit, then, in the complementary, so it should be in the yellow, orange or red end. And you will be asked in some of the problems, in again, problem-set 9 due Wednesday, and you should be able to finish that up pretty quickly tonight after this lecture, and there are some problems on this.
So, for cobalt complexes, you get pretty much the entire range of colors. So I'm just going to end with one more biological example. And here are some pictures of actual colors, and so this is cobalt coordinated to vitamin B12. So one ligand gives you this brilliant red color, another gives you an orange color, and a third ligand gives you a pink color. So you can tell the oxidation state of vitamin B12 by the colors of the molecule. All right. Now you have all the information to finish your problem-set, and that's the end of transition metals. On Wednesday we start kinetics.
Free Downloads
Video
- iTunes U (MP4 - 87MB)
- Internet Archive (MP4 - 85MB)
Free Streaming
Subtitle
- English - US (SRT)