Flash and JavaScript are required for this feature.
Download the video from iTunes U or the Internet Archive.
Topics covered: Free energy and control of spontaneity
Instructor: Catherine Drennan, Elizabeth Vogel Taylor
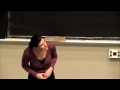
Lecture 18: Free Energy and...
Related Resources
Lecture Notes (PDF)
The following content is provided under a Creative Commons license. Your support will help MIT OpenCourseWare continue to offer high quality educational resources for free. To make a donation or view additional materials from hundreds of MIT courses visit MIT OpenCourseWare at ocw.mit.edu.
PROFESSOR: OK, let's get started. Why doesn't everyone go ahead and take 10 more seconds on this clicker question. It should look familiar. You were pretty split on this question on Friday, so we're hoping after learning a little bit more about delta g of formation, we have at least one direction that wins out here.
OK, great. So now we're up to 85% of you, and hopefully in just a minute we'll be up to a 100%. But if delta g of formation here is less than zero, that means we're talking about a spontaneous reaction when we form the compound. So if it spontaneously forms the compound, that must mean that the compound is going to be stable relative to its elements. So that's kind of the last thing we went over in terms of topics on Friday, and we'll pick up right there today.
All right. Here we have our notes for today, so reminder exam 2 is on Wednesday -- I don't think I need to remind anyone of that. But just please, don't come to this room, make sure that you go to Walker to take the exam. And also if you have any questions or you feel like there's anything you don't understand, I have office hours today from 2 to 4 in my office. Your TAs have also all moved their office hours so they fall before the exam. So make sure you get any of your questions addressed before you're trying to sit there and figure it out in exam time.
All right, so today we're going to pick up where we left off on thermodynamics, so that was talking about free energy of formation. After that we're going to talk a little bit more about the effect of temperature on spontaneity. We touched upon this on Friday, but we're going to formalize exactly in which cases temperature can or can not affect whether a reaction is spontaneous or non-spontaneous.
Then we're going to look a little bit into thermodynamics and biological systems. Two examples that I wanted to talk about were ATP coupled reactions -- those are very important in biology. And also, thinking about the idea of hydrogen bonding, we're going to combine our thoughts on bond enthalpies, and also our ideas of bonding that we had from thinking about covalent and ionic bonds.
All right, so let's finish up first with free energy of formation. So as 85% of you just told me, when we have a case where delta g of formation is less than zero, what we're talking about here is a compound that is thermodynamically stable relative to its elements. So that means we know the inverse as well, which is when we're talking about a case where delta g is now greater than zero, the compound is going to be thermodynamically unstable relative to its elements.
So any time you're looking at delta g of formation, just remember to think about this is just the delta g of a reaction where you're forming a compound. So it should make sense that if it's negative, it's going to be spontaneous and you're going to have a stable compound here.
So we could look at any number of examples, really we could pick any compound to look at, but up on the screen here I'm just putting the compound, the formation of benzene, we've looked at benzene a lot. So benzene has a delta g of formation of 124 kilojoules per mole. Is benzene stable or unstable compared to its elements?
I can't really tell the difference between stable and unstable when you say it, so everyone start at the same time, go.
STUDENT: Unstable.
PROFESSOR: OK, excellent. Benzene is unstable compared to its elements. So that means the reverse reaction here is going to be stable -- or the reverse reaction is going to be spontaneous where we actually have the decomposition of benzene.
So when we're seeing this, when we're seeing that the reverse reaction is spontaneous, a question that might immediately come to us is well, why did we just spend all the time talking about benzene because clearly benzene's just going to break down, right. Why do we form benzene and not have it immediately decompose into its elements. Thermodynamically that is what should happen and it is what does happen, but the reality is that this reaction, this decomposition of benzene is actually very, very slow. It's so slow that, you know we use benzene all the time in organic reactions, we don't see it break down even when we heat it up, is because even though this is thermodynamically a non-spontaneous reaction to form benzene, it's very slow for the actual decomposition of benzene to occur.
So this is just another case, and I'll keep saying this, and when Professor Drennan starts talking about kinetics, she'll keep repeating this as well. What we want to keep in mind is that delta g tells us whether a reaction will happen or whether it won't happen. It tells us absolutely nothing about how long it takes for that reaction to happen. It tells us nothing about the rate of the reaction. We'll keep seeing examples of this, so hopefully no one will be confused by the time we do get to kinetics.
So if we're talking about calculating delta g for any reaction, now we actually have several ways to do it. The first way is very analogous to thinking about delta h for the reaction. We can just look up a table where we have delta g as a formation. So we can take the sum of the delta g of formation of the products, and subtract from it the delta g of formation of the reactants.
We also have another way if maybe we don't have that information available to us. We can also take a look at using this reaction or this equation right here, which is telling us that delta g of a reaction is equal to the change in enthalpy minus t delta s. So that tends to be very helpful, especially when we want to take into consideration temperature.
So let's take into consideration temperature. We've done this a little bit so far, but let's really take a look at some reactions where temperature's going to make a big difference. So the reaction we're going to look at here is the decomposition of sodium bicarbonate, or sodium bicarb here, and it decomposes it into sodium carbonate, plus c o 2, carbon dioxide, and water. So we can think about calculating that delta g of this reaction. I'll tell you that the change in enthalpy, this is actually an endothermic reaction. It requires heat. It's plus 135 . 6 kilojoules per mole.
So let's go to a clicker question and I want you to pick out from several choices which of the changes in entropy seem reasonable to you here. So what would you predict the delta s for this reaction to be? All right, let's do 10 more seconds on that.
OK, so we've got the majority, but not everyone. So let's take a look at why this is the correct answer, that it should be plus 0.334 . If we're going from 2 moles of solid, to 1 mole of solid, plus 2 moles of gas, are we increasing or decreasing the disorder?
STUDENT: [INAUDIBLE]
PROFESSOR: We're increasing the disorder. If we're getting to a more disordered state, then we're going to have a positive change in entropy. We're going to increase the disorder. The only one with a positive delta s is this choice here. So if we switch back to our notes, we can see that, in fact, so what we see is that it's 0.334 kilojoules per k per mole is our delta s, so we can go ahead and calculate our delta g for the reaction, so we're just plugging in our delta h. So our delta h is 135 . 6. And we're talking about to start with let's talk about room temperature. So 298 k times 0.334 .
So what we end up having for the delta g of our reaction is that its 36 . 1 kilojoules per mole. So this is at room temperature. So is our reaction spontaneous or non-spontaneous at room temperature?
STUDENT: Non-spontaneous.
PROFESSOR: Non-spontaneous. All right, but let's take a look at a different temperature. For example, let's look at baking temperature. So if we think about baking cookies, we maybe bake them at 350 degrees fahrenheit, so that would be 450 kelvin -- our ovens are usually set to fahrenheit and not kelvin. So if we think about this reaction that this temperature, first of all let me point out why we would be talking about baking cookies for this particular reaction here. Does anyone know what another name for sodium bicarb is?
STUDENT: [INAUDIBLE]
PROFESSOR: Yeah, it's just baking soda. So this is the reaction that causes your cookies or your cakes to actually rise. So we're producing gas here, and when we're producing that gas when this sodium bicarb decomposes, we're forming these pockets of gas in our baked goods. So if we bake our cookies at room temperature, obviously, they don't bake and they also don't rise because the sodium bicarb, clearly it was non-spontaneous at room temperature, this reaction just doesn't happen.
But if we take a look at baking temperature now, we're going to plug in this new temperature, which is 450 k, and plug this into our delta g reaction. What we find is now our delta g for the reaction is negative 14 . 7 kilojoules per mole. So in this case, we are dealing with a spontaneous reaction, which is good, because this means that when we put our cookies in and we turn it to 350 fahrenheit or 450 k, the baking soda will decompose and we'll got our cookies to rise a little bit.
All right, so one thing that I want you to notice when we were talking about the case with the decomposition of sodium bicarb is that the delta h for that reaction and the delta s, they both had the same sign. And something that you can keep in mind in general is any time that both delta h and delta s have the same sign, it's actually possible to switch from spontaneous to non-spontaneous or vice versa just by changing the temperature of the reaction.
And we can think about this graphically. If we assume that delta h and delta s are independent of temperature, which is a good assumption to a first approximation, in this case we find that the delta g of the reaction is the linear function of the temperature. So that means we can go ahead and graph what we saw for the case of baking soda.
So our first point here was that we saw at room temperature, about 298 k, the delta g was positive, it was 36 kilojoules per mole. We also saw that once we heated it up to baking temperature, we actually had the delta g now at negative 15 kilojoules per mole. So since this is linear, we can actually draw a straight line right through here, and we can think about the fact that we have this temperature here where anything below this temperature has a positive delta g, and anything above this temperature is going to have a negative delta g.
And let's actually think about the fact that this is a line and see what our slope and our y-intercept is going to mean. We can say that delta g is equal to negative delta s t plus delta h, if we want to put it in the formation of the equation for line. So what this tells us is that our slope is going to be negative delta s here, and if we think about our y-intercept, that's going to be the change in enthalpy for the reaction.
So again, what I want to point out is that any time we're at a temperature that's lower than this change in temperature here, we're going to find that delta g is greater than zero, and we're going to be dealing with a non-spontaneous reaction.
However, if we move our temperature up and up and up so we go across the graph this way, eventually we'll hit a point where if we're above that temperature, we'll find that delta g is less than zero, and now we have a spontaneous reaction. So we can actually think about what this temperature is. We can call this T star. This is the temperature at which our reaction switches, whether it's spontaneous or non-spontaneous. And if you're thinking about trying to get a reaction to go, it's very important to be able to calculate what this temperature is. A lot of times in organic chemistry laboratories, they need to heat up reactions -- part of why they do that is kinetics, but the other part is sometimes they need to make a reaction go from being non-spontaneous to spontaneous.
So let's think about how to calculate T star or this change in temperature. So we're talking about this threshold temperature, so we're talking about where delta g is going to be equal to zero, because if we set delta g equal to zero, we know that anything on one side of that temperature is going to be spontaneous, and the other side is going to be non-spontaneous.
So if we do this, we can just rewrite our reaction, delta g equals delta h minus t delta s, and let's plug in our zero for delta g there, and now rearrange our reaction so that we're talking about this threshold temperature. So that T star is just going to be equal to the change in enthalpy divided by the change in entropy. So it's very easy for us to calculate, so let's go ahead and do this for the case with baking soda. And for baking soda what we saw was that delta h was 136 kilojoules per mole, and the change in entropy was 0.334 kilojoules per k mole. And that means if we do that simple division, then what we end up as our temperature star is 406 kelvin.
So basically, what this tells us is if we tried to bake our cookies below 406 kelvin they would not rise, if we bake them above 406 kelvin they will rise because this reaction is now spontaneous.
All right, so this was a case where we had seen that the delta h and the delta s both had a positive value. But let's take a look at what happens when now delta h and delta s are both negative. So we can think about this just by plotting it on our graph again -- we don't have actual values, but we can think about what the sign should be. So if we talk about our zero point where temperature is absolute zero, if we have a positive, or excuse me, if we have a negative delta h now and we're at temperature equals zero, what is delta g going to be? Negative or positive? Yeah, it's going to be negative. So if we have negative delta h and we're at temperature of zero, our s term completely falls out, so we're definitely going to start with a negative delta g.
As we increase the temperature higher and higher, at some point that delta s term is going to become greater than our delta h term, so at some point we're going to flip to where the reaction has now a positive delta g.
So you can draw this into your graphs in your notes where we're going to start, in this case if delta g is negative where delta g starts negative, and then when it hits that threshold temperature, anything above that temperature is going to be positive.
So if we had actual numbers we could plug those into our graph, but we should be able to understand what the general trend is going to be even in a hypothetical case where we're just dealing with is it positive or is it negative.
So what we see is that below this temperature, we have a spontaneous reaction, and above this temperature we have a non-spontaneous reaction. That was flipped from the case where we had delta h and delta s both be positive.
All right, so let's actually summarize what all of our four different scenarios could be if we're dealing with delta h's and delta s's. So to start with, why don't you tell me what you think if we have a reaction where we have a negative delta h and we have a positive delta s, do you think that this will be a reaction that's never spontaneous, always spontaneous, or will this be one of these cases where the spontaneity depends on temperature?
All right, let's take 10 more seconds on this. OK, great. So most of you got it. So let's go back to the slides and think a little bit about why this is. So, this case is always spontaneous, because in this case we have a negative delta h, which contributes to a negative delta g, and a positive delta s, but since the equation says minus t delta s, that means a positive delta s is also going to contribute to a negative delta g. So regardless of what the temperature is, we're going to have a spontaneous reaction. So we say this is always spontaneous -- delta g is less than zero at all temperatures.
All right. So let's look at the reverse case here where delta h is now greater than zero, and delta s is less than zero. Is this always, never, or sometimes spontaneous?
STUDENT: [INAUDIBLE]
PROFESSOR: Nope. So, this is going to be never spontaneous in this case. So for the same reason, because delta h is greater than zero, that contributes to a positive delta g, and delta s being negative also contributes to a positive delta g. So we'll say again, that this delta g is greater than zero at all temperatures.
All right, so now we have a case where delta h is greater than zero and delta s is greater than zero as well. Is this going to be always, never, or sometimes spontaneous?
STUDENT: Sometimes.
PROFESSOR: Sometimes, good. So, even before thinking, you can just remember if the signs are the same, we can have a dependence on temperature here. So what we find is that this is sometimes spontaneous. So we can think about when this happens. Would it be when the actual temperature is greater or less than that threshold temperature?
STUDENT: [INAUDIBLE]
PROFESSOR: All right, so I heard a lot of people say greater than. It's greater than, because when it's greater than that threshold temperature, that means that the delta s term is the one that's going to actually sort of take over, it's going to be at some point greater than the delta h term. The delta h is going to be making the delta g positive. That means it would make it non-spontaneous, but once delta s gets large enough, that's going to override, and now we're going to have a negative delta g only when the temperature is above that threshold temperature.
So similarly, when we see that delta h is negative and delta s is negative, this is also going to be sometimes spontaneous, and specifically, it will be spontaneous or delta g will be less than 0 when the temperature is less than that threshold temperature.
All right, so you should be able to look at any situation where you have or you figure out or you calculate the enthalpy and the enthropy change in the reaction, and you should right away, before doing any calculations, be able to know is this always spontaneous, is this never spontaneous, or is this something where I'm going to need to really take temperature into consideration. And if I do, you can actually calculate what that temperature is going to be where you flip from spontaneous to non-spontaneous or vice versa.
All right, so shifting gears a little bit, let's take a look at a few examples where it's important to think about thermodynamics and biological systems. So there's two things I particularly want to focus on. So the first is the idea of ATP coupled reactions. So this is really important, because when we're talking about biological reactions, a lot of the reactions that take place in our body are actually non-spontaneous, so energetically we figure that the delta g for this reaction is actually positive. So I just show a schematic biological reaction here where we have some molecule that's broken up into two building blocks. So let's say, for example, this has a delta g that's greater than zero. We need to think about how it is that our body can actually make this reaction happen.
And the way that it does this is that it takes the hydrolysis of ATP, and the hydrolysis of ATP is what's called that it's coupled to this non-spontaneous reaction. And when you have a spontaneous reaction coupled to a non-spontaneous reaction, you can potentially drive the reaction in the forward direction. Remember the hydrolysis of ATP is spontaneous, so what we're doing in this case is we're taking a spontaneous reaction where we give off energy, and we're coupling it to a non-spontaneous reaction that requires energy.
So what we will hope is when we add up the total energy changes between these two reactions, if the sum of those two is a negative delta g total, now we can have this whole process move in the forward direction.
So let's take a look at thinking about what we can do in terms of a coupled reaction. The first thing we need to do if we're thinking about using the hydrolysis of ATP is actually calculate what the delta g for the reaction of ATP hydrolysis is, and I picked 310 kelvin because that's the temperature of our bodies. So again, this reaction is taking adenosine triphosphate that has three phosphate groups and hydrolyzing it, so reacting it with water to form ADP plus phosphate plus acid here.
So I'll tell you that the delta h of this reaction is negative 24 kilojoules per mole. This is what we actually calculated in class on Friday. And that the delta s is plus 22 joules per k, per mole, so we can go ahead and calculate what this delta g is here. So the delta g of this overall reaction is just going to be, of course, delta h minus t delta s, and our delta h is minus 24 kilojoules per mole, and we'll subtract 310 kelvin times 0 . 022 kilojoules over k mole. So if we do this, what we end up getting is a delta g of negative 31 kilojoules per mole of ATP.
All right, so again, this is good, we got a negative number, that's what we were expecting. So that means that we will have energy available to use if we hydrolyize ATP and couple it to another reaction here. So what we saw for our delta g, negative 31 kilojoules per mole.
All right, so let's talk about a reaction that is a ATP coupled reaction, and there's just tons of examples of this in our body. One I picked because it has to do with glucose, which we have talked so much about, is the conversion of glucose to glucose 6 p. So 6 p -- p just stands for a phosphate group here, so what we're doing is taking, if we numbered the glucose carbons, this would be carbon number six, and we're putting a phosphate group on carbon number six.
So first of all, why would our body need to do this reaction? It turns out that glucose, we know that we use glucose for energy. Glucose is somewhat apolar, so it can actually move in and out of our cells, because our cell walls, those are very greasy. So what we're actually doing here is we're putting a charged molecule onto the glucose. There's two negative charges in a phosphate group, this is going to make sure that our glucose is now it's very polar molecule. And now that we have a very polar molecule, we're not going to be able to have the glucose move in and out of the cell. So we keep it in our cell by putting this phosphate group on it. That's why we want to do this reaction, but let's think about the energy of actually doing it. And it turns out that this requires energy. The delta g for this reaction is 17 kilojoules per mole.
All right, so that could be a problem if our body had not come up with a way to solve it, which it has, and what it actually does is it couples this reaction here with the conversion of ATP into ADP. And we just calculated that that has a delta g of negative 31 kilojoules per mole. So this means we can think about delta g total, and by total we mean of this overall process here. So that's just equal to 17, and we're adding it to the other delta g, which is negative 31. So we get an overall delta g for this process of negative 14 kilojoules per mole.
All right, so that's one really simple example just to illustrate to you how these ATP coupled reactions work. Some ATP coupled reactions require one molar equivalent of ATP, some require a lot more. But essentially, the idea is the same. We have this reaction that's energetically unfavorable that we couple with an energetically favorable reaction. So, quite literally, this is what we mean when we talk about ATP as being the energy currency for the cell. We spend some ATP in order to get these non-spontaneous reactions to go.
All right, so I also want to talk to a little bit about hydrogen bonding. This also is a topic that deals with the thermodynamics, and it also is related to the ideas that we talked about before in terms of thinking about different types of bonds.
So if we talk about a hydrogen bond, a hydrogen bond, first I just want to be really clear, is not a covalent bond. So this h x bond here is a covalent bond, this is not a hydrogen bond. But a hydrogen bond can form when you have a partial positive on a hydrogen, and you have what's called a hydrogen bond donor atom, which has a partial negative on it, and also has a lone pair. Typically this y atom will be on a separate molecule. And what happens is you have that Coulombic attraction between the partial positive on the hydrogen and the partial negative on this hydrogen bond donor atom. So what you form between them is a hydrogen bond, and you'll notice that I drew it as a dashed line. H bonds or hydrogen bonds are drawn either as dashed lines or as dotted lines, and they're done so to differentiate them from covalent bonds, which we draw with this straight line here.
So let's think about what can form hydrogen bonds. First of all, there's a requirement for what this h x bond can be. It has to be a really electronegative atom in this x here, because we need to we need to form that partial positive on the hydrogen, which means that the atom that it's covalentally bonded to needs to be pulling away some of that electron density from the hydrogen, such that we have a delta positive on a hydrogen atom. Similarly, the same atoms are what can be the y here, so it can either be a nitrogen or an oxygen or a fluorine. So these are the only hydrogen bond donors that can form h bonds.
And we can think about the reason for this and the reason is pretty straightforward. We need to have a small atom, but it also needs to be really electronegative. This should make sense because we need to have this partial negative charge here to have that Coulomb attraction. And the other requirement is that we definitely need to have that lone pair of electrons so it can actually interact in this hydrogen bond here.
So let's take a look at an example of a hydrogen bond, and that's between two water molecules. So let's go ahead and re-draw our water molecules -- they're just kind of randomly oriented there. But let's re-draw them as if they were going to have a hydrogen bond between them. And one thing I want to point out about hydrogen bonds is that they're strongest when you actually have all three atoms in a straight line like this, because it keeps the dipoles the strongest, so the partial positive here and the partial negative here can interact.
So let's re-draw our water molecules like that. So we have our first water molecule here, and let's say we're going to be talking about this hydrogen in terms of hydrogen bonding. Obviously, we have four hydrogens up there that can take place or take part in h bonding, but we're just going to focus on this one here. So that means we want our straight line to be something like this to our second oxygen. So let's draw the hydrogens on this water molecule as well.
All right, so thinking about what the Lewis structure of water is, how many lone pairs do we have on this oxygen here?
STUDENT: [INAUDIBLE]
PROFESSOR: Two lone pairs, great. So let's draw those in, and let's draw these lone pairs in as well. All right, and we're going to be talking about these two atoms in terms of our hydrogen bond, so does this have a delta plus or a delta negative on it? Delta plus. So this is a polar bond here, this o h bond, so we have a delta plus on our hydrogen, and we have a delta minus on our oxygen. So these also have delta plusses, of course, and this also has a delta minus.
So because these two can interact and they're lined up to do so, what we can do is we can draw a hydrogen bond right in between this hydrogen and this oxygen atom here.
So again, I pointed out that, in fact, hydrogen bonds are not as strong as covalent bonds. Since they're not as strong, if you remember thinking about the relationship between bond strength and bond length, would you expect a hydrogen bond to be longer or shorter than a covalent bond?
STUDENT: Longer.
PROFESSOR: Good, OK, it should be longer here. So this is our longer bond because it's our weaker bond. They're not held together as tightly. All right, so this is an example of an intermolecular hydrogen bond, it's between two different molecules. Hopefully, you can also see that if we're focusing on any single one water molecule, we could also form hydrogen bonds between other water molecules with either of these two hydrogens as well, and also with our other lone pair here. So any water molecule can actually form four different hydrogen bonds.
All right, so let's talk a little bit about these hydrogen bonds in terms of their bond enthalpies here. I said that they were weaker than covalent bonds, so let's look at a few comparisons here. So we can look at an h o hydrogen bond versus an h o covalent bond. And what we find is that the bond enthalpies in kilojoules per mole, it's 20 kilojoules per mole for a hydrogen bond versus 463 kilojoules per mole for a covalent bond. So we're not talking about just a little bit weaker, we're talking about much, much weaker for the hydrogen bonds here.
Similarly, if we look at nitrogen, for nitrogen hydrogen bonds, if we have an o h bound to a nitrogen, that's 29 kilojoules per mole. If it's an n h hydrogen bound to a nitrogen, that's 14 kilojoules per mole, and if we're talking about an h n covalent bond, now we're talking about 388 kilojoules per mole.
All right, so again what we see in these cases is that the hydrogen bonds are much, much weaker. They tend to be as low as 5% of what the covalent bond is, so this is actually much weaker than any kinds of bonds we have within molecules, but it's also the strongest type of bond that we can have between two different molecules. And one thing that you find as in the case of water, is when you have lots of hydrogen bonds between molecules it changes the property of, for example, water here. It makes the boiling point much, much higher than you might expect if you looked at the other properties, because you have to actually break apart all of these individual hydrogen bonds, and even though it's not that much energy for one individual hydrogen bond, once you get a huge number of hydrogen bonds, you're talking about huge energies here.
So let's look at some examples of where we see hydrogen bonds in biological processes. First thinking about proteins, they're absolutely hugely important in proteins. And in proteins, we're talking about a molecule that's so large that you don't just see h bonds in between two different molecules, what you actually see is what's called intramolecular hydrogen bonding, so hydrogen bonding within the protein molecule itself. And hydrogen bonding is incredibly important, it, in fact, is the shape of a protein, which I'm just showing an example here, histone deacetylase, and the histone deacetylase molecule actually has just countless hydrogen bonds in here, and the hydrogen bonds largely govern the shape of the molecule.
So, if you're thinking about any protein that has a shape, so for example, we see these ribbon structures here, they're called alpha helices, you can see some arrows, which are just like a sheet structure or beta sheets, that actual shape is stabilized and formed in the first place by just many, many hydrogen bonds within the protein being in a correct orientation that allows for this particular shape to be stabilized. And we know that shape is so important when we're talking about proteins, and that's because the actual shape of the protein governs the interaction with other proteins or other small molecules.
The shape of the protein is very important in terms of positioning the specific atoms that are involved in the chemistry that the enzyme carries out being in the correct position. So hydrogen bonds are very important in terms of proteins. We can also think of them in terms of sugars or polysaccharides. This is a nice dramatic example, thinking about the structure of a protein is a very microscopic way of thinking about hydrogen bonding.
If we want to take it to the macroscopic level, we can talk about h bonding in trees. So if we're talking about trees, trees are made up -- a major part of a tree is the polysaccharide cellulose. So that's shown right here. Cellulose is a chain of glucose molecules linked together. It can be as many as 1,000 or more glucose molecules linked together covalentally. But what actually happens to those individual chains is that if you look at this picture here, all those dotted lines are actually hydrogen bonds. So the individual molecules are in a much larger chain of hydrogen bonded molecules and they're incredibly rigid. You have so many hydrogen bonds that now it takes a huge amount of energy to break all of these.
So that accounts for why wood is such a hard, solid material. And also, I mean for any plant, and tree is the most dramatic, the structure of a tree, the macroscopic structure, can be explained by hydrogen bonding. It's hydrogen bonds that keep all of those molecules together in the forest and in trees.
All right. So, that's proteins, that thinking about sugars. We can also talk about the importance of hydrogen bonding in DNA. So if you think about the characteristic DNA double helix, hydrogen bonds, we have in a double helix we have two strands of DNA that you can see are intertwined here. And those two strands are actually held together by hydrogen bonding. So specifically, it's hydrogen bonding between what are called complimentary bases within the DNA.
So, for example, if we look at guanine and cytosine here, you can see that there's several places, when they're lined up like this, where we can have hydrogen bonds. So in terms of thinking about how these two molecules are lined up, how many h bonds would you expect between these two bases? Yeah, so it's lined up that there are three that can form here. So we have an o h hydrogen bond, an h n, and then an h o hydrogen bond that can form.
So the other side of complimentary based pairs are AT base pairs, and if you look at the way these are lined up here, how many hydrogen bonds can form between A and T base pairs? Yup, so it it's two that we can form here. We have one between the h o, one between the n h, and you see that we've lost this nitrogen, this n h group here, and first of all this would be too far apart anyway for hydrogen bond, but we can't have a hydrogen bond form when we have a carbon h. Remember, it has to either be a nitrogen or an oxygen or a fluorine because those are the atoms that are going to pull away enough electron density from the hydrogen to give it a partial positive charge that it needs.
So in terms of thinking about DNA, this is a really neat case to consider the actual thermodynamics or the bond enthalpies of the hydrogen bonds, because, of course, does not always stay in it's double helix -- when we're talking about transcription, we actually need to unzip or separate this helix into its two strands, so each individual strand can be copied.
So it's really important that hydrogen bonds are strong enough to hold the DNA double strand together, but that they're not so strong that when you actually pull the hydrogen bonds apart to open up the double strand, that you actually, you don't want to break all of a covalent bonds in DNA as well.
All right, so that's all we're going to say in terms of thinking about thermodynamics and biological systems. Hold on a sec, we have plenty of time left. I want to go over a few clicker questions. I didn't want to give you too long set of notes, because we're switching over to Professor Drennan's going to start lecturing on Friday, so I thought maybe I shouldn't have to have her finish up with my notes.
So, Professor Drennan will continue talking about thermodynamics and thinking about equilibrium, and then she'll transition that into talking about kinetics. So that's actually what's going to start happening after the exam on Wednesday. But let's take a look, since we do you have a little bit of time left, at a few clicker questions just to make sure everyone has caught what we've gone over so far. And a few reviews for the exam, and I will have this clicker question or one of the ones following, be a clicker question quiz, so make sure you are still answering these questions here.
So the first one covers what we went over today, so I want you to tell me and try to not look back in your notes for this, for a reaction where you have a positive enthalpy and a negative entropy change, would you expect this reaction to be never, always, or sometimes spontaneous? So let's take 10 seconds on that, that should be pretty fast.
OK, excellent. 90% is very good. So this should never be spontaneous, because we both have the entropy and the enthalpy term contributing to a positive delta g.
All right, let's shift gears to a couple of questions that are review for the exam. So hopefully, these will all be very straightforward for you now. So I want you to think about bond lengths here, and tell me which molecule contains the shorter nitrogen oxygen bond. So, we're comparing n o minus 1, and n o 2 minus 1. So if you wanted to get started on this problem, what's the first thing you should do? Yeah, draw some Lewis structures. So that might be a good place to start in thinking about this.
So I'll do that up here as well, but don't look if you want to try it on your own. Remember, the quiz points come for answering, not for getting it right. So try doing it on your own here.
All right, let's go ahead and take 10 more seconds on this one. OK, hold on. Don't show the correct answer. You know we're going to re-poll and give you a little bit more time to see if we come to a consensus here. So let's take another 30 seconds or so on this while I finish drawing these up here.
OK, good. So we've got 81% have it correct that in the n o minus 1 bond here, this is going to be a double bond.
STUDENT: What if [INAUDIBLE].
PROFESSOR: No. It would be a double bond here. So, if you follow just the Lewis structure rules, and you go ahead, you'll find that we end up having four bonding electrons available. So you can just go ahead and plug those in, and you end up with this many left. If we had a triple bond, then we would end up having more bonding -- or we would end up using more electrons than we have available for bonding here.
All right, so we have a double bond in the case of n o. What is this bond that we have here? Yup, so it's actually a 1 . 5 bond. What is this bond that we have here? STUDENT: [INAUDIBLE]
PROFESSOR: All right, so we have two 1 . 5 bonds in this case, how come these are 1. 5 and not, for example, a double bond?
STUDENT: [INAUDIBLE]
PROFESSOR: Great, so that's the key. Even though we have double bonds in each of these structures, in this case here, we have resonance, so it's turns out to be, in fact, two 1 . 5 bonds. So since this is the double bond, it's going to be the stronger bond. Since it's the stronger bond, it's also going to be the shorter bond. So make sure you can make those relationships between bond strength and bond length and thinking about if you have resonance or you don't have resonance in a particular situation.
All right, let's try one more clicker question here. We'll have this be the last one for you. So this is one that hopefully you'll all get right, because we've gone over this again and again both in class and recitation. So let's talk about the hybridization of the specific carbon and oxygen atoms in ATP, so I want you to go ahead and tell me what these hyrbridizations are for these two atoms.
OK, let's take 10 more seconds here, get those final answers in. OK, good, so 83% of you got this. Let's take a look at why. So carbon a is bonded to three things, but it is bonded to no lone pairs, so we need to have it be three hybrid orbitals, so it's s p 2. And oxygen is bonded to two atoms plus two lone paris. So we need four hybrid orbitals or s p 3.
All right, so this was your quiz question, so as long as you answered it, you got your quiz points for today. And you can get going a little bit early today and finish studying for this exam.
Free Downloads
Video
- iTunes U (MP4 - 98MB)
- Internet Archive (MP4 - 98MB)
Free Streaming
Subtitle
- English - US (SRT)