Flash and JavaScript are required for this feature.
Download the track from iTunes U or the Internet Archive.
Description: This lecture introduces the development and differentiation of the spinal cord following gastrulation.
Instructor: Gerard E. Schneider
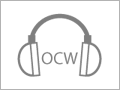
Lec 7: Spinal cord developm...
The following content is provided under a Creative Commons license. Your support will help MIT OpenCourseWare continue to offer high quality educational resources for free. To make a donation or view additional materials from hundreds of MIT courses, visit MIT OpenCourseWare at ocw.mit.edu.
PROFESSOR: I wanted to say a few things about the end of, what was meant to be the end of class six. It was chapter seven in the book. It was meant to be an overview of the forebrain.
And at the end here I was talking about the-- we got through the limbic system, and then I wanted to say a little bit about the neocortex. So this picture here, this gives a little outline meant to show the addition of neocortex. Here is a slide where I'm showing that the limbic system is, one way to define it is by connections to the hypothalamus.
But now when we get to the neocortex, the first topic that we always think of neocortex is as so important in learning. And I want to point out that it was in the early olfactory system, long before there was any neocortex, there evolved these two types of learning, that have remained the two major types of learning, that do involve neocortex in mammals, including the human beings. Two different kinds of learning.
So what are these two different kinds of learning I'm talking about? You can think of them in terms of the structures that are involved, or you can give a name. The types of learning. You know what I'm talking about, any of you?
Remember I talked about the early evolution of olfaction, and how the connections were with this ventral-most part of the striatum, the corpus striatum. And I said that the important thing about those connections was that they were plastic, they could change.
That's how animals learn to like certain smells and not like others. They identify objects that way. But they did another thing, too.
Different regions they went to smelled different. So without any vision, there were two kinds of learning that were possible. Places that were good or bad for them, according to the feedback they get, and the objects in those places that could be good or bad to eat, for example. They also, of course, could smell predators. And that's still true in many mammals.
So this is the way I summarized it. Learned object preferences with identification of objects according to whether they're good or bad. And place learning, identification memory of good places and bad places.
The first one is striatal dependent. And that remains true even though the habits formed aren't dependent so much on olfaction. In primates they're mostly dependent on other senses, but olfaction is still important.
The other is place learning. And that has become critical for what humans usually think of when they think of memory, long-term memory formation. And that will be a theme that will recur in the class.
And what is the part of the brain involved here? We always think of hippocampus. But in many animals, long before you could really identify something that looked like a seahorse or a hippocampus, it was part of the pallium. It was the medial pallium.
In a picture like this, it's this part here. It's meant to be medial. It's a little hard to show medial and lateral in a picture like this. But it's part of the limbic system, connected to the hypothalamus.
And we're going to discuss later why that came to be true. How that evolved. There are good reasons why we became so dependent on parts of the limbic system for our memories.
So now, with neocortex expansion there were new sensory pathways that became prominent. They were more rapidly conducting than the spinal reticular pathways and the spinal thalamic pathways in sending information to the forebrain.
Now we're talking mainly about pathways to neocortex. And here I'm just showing the addition of cortex. And then here I've altered this diagram here to show neocortex up here. There's the medial pallium. So I made it look like a hippocampus.
So that's part of the limbic system, which is all of this in the diagram. And this is really also limbic, but it's olfactory. The olfactory parts of the limbic system. They're limbic because they're closely connected with hypothalamus. In fact, many animals actually have direct olfactory connections to the hypothalamus.
So now the two pathways. I don't know why, but students always find it difficult to memorize this. We talked about spinal reticular, we talked about spinal thalamic. Now here is another system that has a number of branches, but many more of its axons go all the way to the thalamus.
So it's a pathway to the neocortex by way of the thalamus. And I've shown here that the first part of it is the dorsal columns. They are actually axons of primary sensory neurons. So here they start, out on the skin. Specialized end organs like Pacinian corpuscles, sensitive to touch and things like that, in the skin.
There's its axon. It does branch. It connects in the spinal cord, too. But it ascends all the way to the top of the spinal cord.
And some anatomy texts say caudal end of the hindbrain. It's a little bit arbitrary where you mark that division. I follow [INAUDIBLE]. He puts it at the very top of the spinal cord. That's the dorsal column nuclei.
We'll be talking about that very soon. We'll see the dorsal column nuclei, representing all different parts of the body except the face. But in fact, the face is represented there too, and I will show you how.
But that is the first synapse in the pathway. So it conducts rapidly. They tend to be all myelinated, fairly large axons.
So this pathway now, from the dorsal column nuclei, decussates right here and ascends in a pathway through the hindbrain and midbrain that we call the medial lemniscus, the medial ribbon. So that goes directly to the thalamus.
There are a few branches that go into reticular formation, but the main pathway goes right to the thalamus. And those thalamic cells connect to somatosensory neocortex. That's how our somatosensory cortex gets its major input. It does get input through the spinal thalamic pathway, and even a little bit from the spinal reticular also. But at least for fine touch, very discriminative touch, especially the touch that we use when we're moving our fingers over something, very dependent on this pathway.
So here is the top view. It shows the same thing. The primary sensory neuron and its axon terminating. If this is in the lumbar enlargement, so lets say it's coming from the foot, it would go to the medial dorsal column nucleus. There's two nuclei there. The medial one represents the lower body, and the upper one represents the arms and chest.
And there's the axon that decussates. The axon of the medial lemniscus starts from the dorsal column nuclei, crosses over, goes to the thalamus. So it's a crossed pathway. So that all evolved after the crossing evolved. Which was admittedly very early, very early chordates.
But if we look at the most ancient ones, we look at hagfish, we look at sea lamprey, they don't have any pathway resembling this. In fact, most mammals don't have much of a pathway that's like this one. It's the mammals this becomes prominent, and other advanced vertebrates like birds.
And now, along with that, you get descending pathways coming down from those somatosensory regions. And one of the somatosensory regions became more specialized for controlling fine movement. That was the motor cortex.
So I'm showing it here on the side view. And I'm depicting two neurons for a good reason. Because I want to point out that the somatosensory, what we call somatosensory cortex, and motor cortex, both have pathways to the spinal cord.
Here's the one you're probably most familiar with. It comes from motor cortex. Note that there are branches to the striatum, to the thalamus, to the midbrain, to the hindbrain, especially to the pons. But it goes all the way down to the spinal cord, where most of the axons and interneurons are in the ventral horn. A few of them, as I showed here, go directly to the motor neurons.
Now the somatosensory cortex has similarly descending pathways all the way to the spinal cord. But these terminate in the dorsal horn, the part of the spinal cord that gets the sensory input from the dorsal roots. We'll show the adult. We'll be talking a little more about that very soon.
Here shows that that, of course, has to be a decussating pathway, too. Because we know that the opposite side of the body is represented. If this is the left side, it's represented on the right side of the brain.
So that pathway way decussates too at about the same place the medial lemniscus decussates. At the caudal, that's a very dramatic place in the brain, between hindbrain and spinal cord, because of the major decussations that occur there just above the dorsal column nuclei.
I mentioned birds have this too. You have a structure in birds, particularly prominent in the more advanced birds like owls and other raptors, but many birds have this. The word wulst in German means a bulge. And it does form a bulge in the hemispheres of these animals.
And they have a motor wulst just like our motor cortex. It projects directly to the spinal cord. They do have somatosensory wulsts and visual wulsts as well.
They also have a very large subcortical region that has connections also like neocortex, just like the wulst does, but it doesn't require cortex at all. It's actually subcortical. It's called the nested pallium, or the nidopallium. We'll mention that a few times. Just so you know something about other companions, advanced vertebrates.
Now let's just mention very briefly what characterizes the sensory and motor functions of the neocortex and what other types of functions depend on neocortex. Think of neocortex as adding to sensory processes. Acuity, both sensory and motor, fine movement control, find discrimination of objects. But it also made it better at objects could be separated from the background more easily.
So it's not just a mishmash of stimuli they're responding to, but they respond to specific objects. So that means they have to form images of those objects. And that's something neocortex does.
And I think the most unique thing about neocortex-- just that fine control, the acuity, sensory-motor, that's just sort of a quantitative improvement over what lower structures did. But this is something really unique. It adds the ability to anticipate and plan.
Anticipate stimuli, we do that with image formation. Imaging depends on the posture of parts of the neocortex. And also we plan movements. We don't just react. That uses an internal model, a kind of simulation of the world. It depends on those images as well.
So those are really the unique parts. I put review slides at the end of this chapter that you should look at. I want to get on here with the main topic of the day, formation of the neural tube in the embryo-- how central nervous system development begins.
And we'll focus on the spinal level. That is where the neural tube first fully forms. But the entire central nervous system begins as a tube.
Remember, it all evolves, actually, together. So we're going to do the embryology today. And next time we'll be able to get to the adult spinal cord, and then also the autonomic nervous system.
These are the stages of development of a nervous system. After the egg is fertilized you get the formation of a morula, a clump of cells. That becomes a blastula, which is a hollow ball of cells. Now it's got fluid in the middle.
Then a major step, gastrulation, where the organism, instead of just this hollow ball of cells with fluid in the middle, becomes shaped like a doughnut. The formation of the alimentary canal-- mouth at one end, anus at the other. That forms very early. We'll see pictures of that. And then finally neurulation, the formation of the nervous system, the major thing we want to talk about.
Now, Lewis Wolpert, in his very interesting little book, Triumph of the Embryo, when he revised it, other authors joined him and it became a more standard embryology book. This is also very interesting. All of the things that I found in Triumph of the Embryo are still there, but he really simplified it and talked about the major principles. And one of the things he laid out was the cellular events, what are the cellular events that characterize development of the nervous system? What are they?
Well, contraction. Parts of the cell could contract. What does that involve? In involves contractile proteins, just like we have in muscle. Cells have that also, to some degree, in development. Contraction.
Well, cells have to, in order to form organized tissue, cells have to be able to stick to things, stick to each other to form these little processes that can adhere to something. So they show adhesion. And the adhesive properties through cell adhesion molecules in the membrane, which can change during the course of development. That's a big part of development, selective adhesion and changes in adhesion.
And then cells can move. How do they move? So we'll be talking about that. And finally, of course, growth. And by growth, often an embryologist is talking about growth by proliferation of cells, mitosis. It also means growth and increased size of cells. But the main factor is increased numbers of cells.
So here's the pictures that Wolpert uses. From a single fertilized egg here, to the morula. It looks like a raspberry. And here it becomes the blastula with fluid in the middle.
And then note some cells have moved to the interior. And there's more contraction at one side, the outside of the cell, than on the inside. And that leads to invagination that you see beginning here. And then look what happens.
These little processes extend out. Those little filaments are called filopodia, filamentous feet. They extend out, and they actually reach the other side, where they adhere. So now you have adhesion.
You have both of these processes we just talked about are happening, contraction and adhesion. Movement-- notice the movement that's being caused by the contraction, contractile proteins in those filopodia. So it pulls this side of the embryo right across.
And that causes changes that cause an opening to form between here and here. The cells continue to move to the inside. But that process from here to hear, or actually all along the bottom, is called gastrulation. This is just seen in the sections.
This shows us one of the cells, how complicated they can be with their various extensions and movement. Because if this was all there was, we'd have one layer. That outer layer is the ectoderm.
But cells move inward. They move to the interior. And that becomes the mesoderm and the endoderm. Mesoderm forming muscle and skeleton, and the endoderm forming the internal organs.
So then none of this gives you a central nervous system. How do we get a central nervous system out of that? So we need to talk about neurulation, the formation of the neural tube. And then proliferation of the cells, migration of cells, and differentiation of cells.
By differentiation we mean the cell changes so the cells look different from each other. They differentiate from each other. They form dendrites, they form axons, if they're neurons. If they're glial cells they also form processes, depending on what kind of glia they are.
So these are the questions I'm asking. First of all, what is the notochord? We've heard that term used before. You should remember what it is. Some of you might.
What is the notochord? It's not in all animals. It's just in all animals that we call chordates. So what is it? The notochord. Yes?
AUDIENCE: The cartilaginous tube that becomes the--
PROFESSOR: Yes. Tube may not be the most accurate. It's cartilaginous for sure. What is it?
AUDIENCE: It's beneath the neural tube.
PROFESSOR: But wait. It precedes the neural tube. But where in the organism? At least, what does it become in the organism?
AUDIENCE: In the back.
PROFESSOR: The back. Exactly. It's always along the back. At least what's going to become the back. Maybe that's how it becomes a back. The notochord is at the surface, one side, that becomes the back.
AUDIENCE: Does notochord become backbone?
PROFESSOR: It doesn't become the backbone, but the skeletal elements form around it. There are induction processes. Molecules coming from the notochord exert really powerful changes. First of all in the formation of the nervous system, but then the formation of the skeleton as well.
Who discovered this phenomenon of induction of the CNS? Now, we know now that the inductive process, the notochord induces the formation of the nervous system. But how was it discovered? Who discovered that there's some kind of induction that's happening?
Because if you move things around, you can get nervous system forming differently, or in different places. Remember the names? There were actually two people, but only one of them got the Nobel Prize for it.
His name was Hans Spemann. Spemann got the prize. But the discovery was made in his lab by his student, a young woman named Hilde Mangold. Why didn't she get the Nobel Prize?
Unfortunately, they don't give Nobel Prizes to someone who has died. She died in a kitchen accident when she was only 26 years old. But she should be remembered just as Spemann is for the discovery of induction of the nervous system.
And then we want to know what neural crest cells are. You've got to know something about the neural tube formation to be able to answer that. I should have a piece of paper here. I didn't bring my piece of paper.
You just take a piece of paper and push at the two ends. What happens? It will indent, right? And you can get it to indent in one place. That's what happens to the nervous system here.
Here's a sheet of paper. It's one cell thick ectoderm. And that is the notochord. This is just part of the ectoderm, the part that will become the back of the organism.
There's the notochord. Then there's an influence from the notochord. Note here I show cells getting thicker in this region. That's what we call a neural plate. The cells are already determined now. Which ones are going to become nervous system and which will remain other parts of the ectoderm, like the skin.
And then, because of differences in contraction, despite during gastrulation earlier, you get an endogenization. So it doesn't really happen because somebody pushed the sides like a piece of paper. But the dynamics are very similar.
So it starts to form a groove along the back of the organism. So think of it in three dimensions. That's why I like you to think of a piece of paper. If we push in, it's gonna form a groove all along the back of the organism. There's the notochord. The molecules emanating from that notochord are what's causing these changes in the ectoderm above it.
And note I show two little bulges on the sides. That is called the neural crest. And it's very different. Because as these two edges come together so you end up with a tube, these cells don't become part of the tube. They stay on the outside.
It's those neural crest cells that form the peripheral nervous system. All the cells of the peripheral nervous system come from the neural crest at this level of the nervous system. Now, there are cells that don't come from neural crest in the head region.
There they come from both neural crest and from what we call placodes. It's thickening of the ectoderm that happens due to other inductive influences. Not the notochord now. It can also come from peripheral nervous system. But here in the spinal cord, it all comes from the neural crest.
And then there's a few names you should learn. When this tube forms, note I show here the tube is already getting a little thicker. There are cells proliferating there. They proliferate in the sides of the tube, not at the very top and the very bottom.
The very top is called the roof plate. It remains one cell thick. And the bottom is the floor plate. It also remains one cell thick, right to the adult.
But the walls get thicker and thicker. And in development the ventral part closest to the notochord tends to proliferate a little bit faster. That's where the motor neurons will form.
So we call the upper part here the alar plate. And the lower part the basal plate. And little groove separating the two is called the sulcus limitans, the limiting sulcus. And we'll see pictures of that as we go on.
Let me show you some pictures of it. We mentioned this already, the discovery of induction. Where does that closure begin? Remember, it's a groove.
It's a neural groove all along the back of the organism. And then it forms a tube, as you see in this picture. Well, where does this first begin? So I show you this picture. It begins sort of in the middle.
This is the head, this is rostral. This is the caudal end here. That's where the neural tube is first closing. So if we go further back or further forward, it would look like this. It would be a neural groove still.
So it starts to close. That region corresponds to the cervical region of the spinal cord, the upper part, or the most rostral part of the spinal cord. That's where development is a little more advanced. That's where the neural tube first closes.
So the last region to close, as you can guess, it sort of zips up this way and zips up caudally, the very tail region here. The last part to close. And similarly, the most rostral part of the CNS, the last part to close.
So if some abnormality occurs during early development, and it does happen, you can get a failure to completely close. When it doesn't close caudally here, you get a condition known as spina bifida. That can be corrected surgically.
But when it fails to close rostrally, there's a failure of the forebrain to develop. You call it anencephaly. Basically it means without a brain. They do have some brain.
They have the brainstem. And babies that are born that way don't live very long. It's impossible to repair that. They've been studied a little bit in their brief, very brief lives.
Let's take a look at that happening. These are interesting animations that you can actually find on the web. If you look at the cross section up here-- don't take notes now, look up here. Look up here. Look at the screen or you're missing the main show.
You see the neural tube in the 3D picture there closing. You see what's happening up there. The black dots are the neural crest cells that are staying on the outside. They don't get incorporated. The tube closes without them.
The red is the mesoderm, mostly muscle tissue there. And the vertebrae are forming around the neural tube. So that's where you find the peripheral ganglia of the sympathetic nervous system. And there's the dorsal root ganglia.
What are these out here? They're the neural crest cells. I didn't mention those before. They're migrating into the skin, mostly melanocytes, the pigmented cells in the skin.
Rather than look at that again, I want to show you an actual photograph of neurulation in the clawed frog, the Xenopus. It happens very fast, so we'll look at it several times. If you just focus on one of these, you see the whole thing because they've speeded it up so much.
With the formation you don't really see the neural plate, but you see the neural groove forming and you see it close. It zips up rostrally and caudally. And then the embryo turns a little bit so it looks a little bit more like the end of this one.
You can find those, if you do some searching. You can find them online. This is just a picture of that early stage, taken with the electron microscope when they used a method called freeze-fracture.
They freeze it solid. And then if they could get it to break along this line, you can see individual cells in both the CNS there in the neural tube, and the mesoderm and the ectoderm. And then you see the endoderm down here.
And this just shows what it's like in humans at particular stages, 22 and 24 days after conception. I picked this because it's right after the neural tube starts to close. In the hamster, that I've used a lot, it closes on the eighth day after conception.
So it can happen in some species very, very fast. Of course, they're much smaller too. It tends to be slower in the larger animals.
So what is this term, sonic hedgehog? We're not going back to comparative neurology here. It's the name for what?
AUDIENCE: [INAUDIBLE]
PROFESSOR: It's a particular molecule, sonic hedgehog. It's just the name for a protein, a molecule. It actually has a number of roles in the formation of the nervous system. Where's it come from in this process we're starting right now? I told you before, where the induction comes from.
The notochord. Sonic hedgehog is generated by the notochord. It induces the formation of the nervous system, and does other things too. And if we look at this picture, they're coming-- these would be where the molecules are.
Of course, they're probably going all around. I'm showing the ones that are in a concentrated way affecting these cells above it. But still coming there. And it's affecting this lower part of the cord.
It serves as a ventralizing influence. It makes that ventral part of the neural tube-- see, they're still here. It makes the cells here form differently than the cells up here. Without sonic hedgehog and its ventralizing influence, you wouldn't get to form early differentiation of motor neurons.
What about the dorsal part? There are inductive influences on this part, the part that came from way out here. It forms the dorsal part of the cord. Those inductive influences came from the ectoderm. And I mention it here.
The dorsalizing factors are secreted by the ectoderm adjacent to the neural tube. The main ones are this BMP-4 and 7. BMP actually means bone morphogenetic protein, because it has a role in bone formation as well. And now we're talking about its influence on the nervous system.
So it induces changes in the dorsal part of the cord. It causes those cells to develop differently than the ones ventrally. That leaves a lot of things unexplained. But at least it was a start in looking at how the nervous system develops.
Now I want to talk about cell division. What are the two main types of cell division that I described in this chapter? There are differences in cell division that make a huge difference in h how the nervous system forms. Symmetric and asymmetric.
And for that, you need to look at a picture like this. This is the cell cycle. The cell here moves away from the ventricle. But when I say the cell, I really mean the nucleus of the cell. Because it's attached to the pia out here, to the ventricle here.
But it moves away from the ventricle in order to synthesize its DNA. It moves towards the ventricle in order to undergo mitosis. And it can undergo mitosis with a symmetric split or an asymmetric split.
When it splits symmetrically, it tends to split along a line that's at right angles to ventricular surface. And when it does that, the distribution of proteins in the cell-- there's two proteins in particular-- tends to be pretty even in the two daughter cells. So the two daughter cells will behave pretty similar. Basically, they remain stem cells, they keep dividing.
But when it splits asymmetrically, you end up with a very uneven distribution of those proteins. Notch and numb they're called. And now you get the two cells behaving differently. One remains a stem cell. It keeps dividing.
The other one becomes post-mitotic and does something very differently. It migrates away. And in many cases the migration is simply, like over here on the cell cycle, the nucleus just moves away from the ventricle. It can be doing that. The attachments can remain. Cajal saw things like this.
These are the way they look in Golgi in many cases. You might have been looking at two cells here. But in some cases they might be cells with two nuclei.
Here's a cell that's lost its external process. All of these in here, they've regained the attachment to the pia. And when the nucleus just moves further and further from the ventricle and never returns, we say it's migrating. We say it's migrating by nuclear translocation, the first type of migration.
Here's his picture of the chick spinal cord on the third day of incubation. Chicks grow very fast. They're a favorite among embryologists because you can cut a little window in the egg and you can look at these things happening.
And note that some of the cells are right near the ventricle. But all of them that he shows in this particular picture are attached to the pia. So it's really similar to roof plate and floor plate there. It's just that this has a lot longer here and there's more of them.
Well, if you look at other day three pictures, let's just look at that right now. Here's another day three picture. Golgi is selective. Sometimes you're getting more advanced cells, when you're looking at developing animals, sometimes you're getting the early developing cells.
But you see on that same day, perhaps later in the day, he was able to identify ventral and dorsal roots forming. How did he do that? By recognizing something that he was probably the first to recognize. And he named them.
The active growing tips that we call a growth cone, processes extending out. And when he saw that happening here, and he looked at them a little older, he would see the growth cones further away. He could see that an axon was growing, forming a ventral root. He started seeing that in day three of the chick.
He also saw growth cones begin in the developing spinal cord. And here he saw some of those cells of the neural crest that weren't in the CNS, he saw them with two growth cones.
One growing out towards the periphery. Another one growing right into the neural tube. And that was Cajal's work.
So here I'm asking how did neural scientists come to know that there at least two major modes of cell migration in the embryonic [INAUDIBLE]? Here's what happened.
I just described nuclear translocation as a type of migration, like this picture here. When these cells are moving like this one, it's moved very far, it's moved way out towards the pial surface. It never comes back because it's not in the cell cycle anymore, yet we say it's migrated. And eventually it loses its process. It usually uses both of its attachments, to the pia on the one side and to the ventricle on the other.
That had been described, had been seen by Cajal. It was described by Kent Morest, who was at Harvard and then moved to the University of Connecticut. And then a man named Pasko Rakic, also at Harvard, but then he moved to Yale, he worked on a different part of the brain. He worked on primates.
He discovered evidence that in primates that is not how cells migrate. They attach to a glial cell that was elongated. And they used the glial cell sort of as a ladder, and they moved along it.
So he said that's the way cells from the central nervous system migrate. And there actually became a sort of a conflict between these two views. Which was really silly. Because as you might expect, both occur.
And I want you to know how that argument was settled. By proof that nuclear translocation is not just in the spinal cord, but right in the brain. It can be the major mode of movement.
Now we know there's other kinds of cell movement too, among other substrate factors. But we're just gonna talk about this. And we'll talk more about guidance by radial glial cells much later when we talk about development of cortex.
But let's talk about a study by these two people, Morest and Valerie Domesick, who was a student [INAUDIBLE] here at MIT that went to work with Morest. This will be just briefly the last topic.
They were studying a cell in the tectum that was very interesting. Because if you look at the adult, you would see the cell and its dendrites here near the surface of the tectum. The initial part of this axon had this peculiar bend that looks like the crook in a shepherd's staff. So it was called the Shepherd's Crook Cell. That was the name of it.
They looked at earlier and earlier periods. They kept seeing the crook. But they found the period where the axon was just beginning to form. It had the peculiar bend. There's the growth cone.
And then they saw that at that stage the cell body was way down here, near the ventricle. The cell body hadn't moved yet. It hadn't migrated.
And yet the axon was already forming. So you have to realize that the cell extends all the way from ventricle to the midbrain surface here. The axon is forming here, long before the cell body is there.
And then the cell body is moving, as you see here. And finally it gets to the point where the axon originates. They looked at many embryos closely spaced in age and they saw all these changes.
And they reported it at the American Association of Anatomists meeting, where a group called the Cajal Club met. It was a favorite meeting of neuroanatomists at that time. It was before there was a society for neuroscience.
But their talk was so convincing, and Rakic was there. He agreed afterwards, OK, there's gotta be at least two kinds of migration. Because I know that that's not how it happens in the neocortex. So that's the story of how we became more certain that there were two different kinds of migration.
So that's where we're gonna stop today. We've already talked about this. I guess we've finished, except for review.
So I'll post these slides. You can go over them. And we'll be able to talk about the next chapter about spinal cord on Friday.