Flash and JavaScript are required for this feature.
Download the track from iTunes U or the Internet Archive.
Description: This lecture concludes the discussion of specializations in the evolving CNS, including sketching the brain and basic pathways of ancestral mammals.
Instructor: Gerard E. Schneider
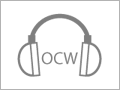
Lec 6: Specializations in t...
The following content is provided under a Creative Commons license. Your support will help MIT OpenCourseWare continue to offer high-quality educational resources for free. To make a donation or view additional materials from hundreds of MIT courses, visit MIT OpenCourseWare at ocw.mit.edu.
PROFESSOR: Two things I want to do today-- just briefly go through chapter six, which is about brain specializations. It was meant to be sort of an interlude. There's a little bit of a break before we get into some of the forebrain connections. But these all involve specializations because of the evolution of head receptors and the resulting expansions of the brain that have occurred. And we start with electroreception in some fish that's led to this enormous expansion of the cerebellum in the mormyrids.
And my first question here was, what cranial nerves carrying information from electroreceptors in the fish that have electroreception? And also, why is it needed? Why don't they use vision?
AUDIENCE: [INAUDIBLE].
PROFESSOR: They're cranial nerves, that's right. But what cranial nerves are they?
AUDIENCE: [INAUDIBLE].
PROFESSOR: There's actually up to six of them. And what are they called? The lateral line nerves. Because fish have these receptors along a line along the side of their body. They also have mechanical receptors there. There's a mechanical lateral line row of receptors also, but we're concerned here with the electroreceptors.
So what are they doing? Why is it so important? Why aren't they just using vision? Yeah, go ahead.
AUDIENCE: [INAUDIBLE].
PROFESSOR: Yeah, because they can't see. They're living in muddy waters. They thrive in those waters, but they've got to be able to find things. They've got to be able to find prey if they're feeding on them.
So what are they detecting? There's two kinds of electoreception. Let's just deal with one of them-- the fish that generate an electric field around their body. It's not a big electric field. There are a few of them that can generate such a big jolt of electricity that they can stun prey, but we're not talking about those. We're just talking about them that use it as a sense.
They generate an electric field, but they also detect the electric field all around the side of their body. So any disturbance in that field they can detect. So all that computation involved in this specialized brain here, the cerebellum-- this is the area in one of the mormyrids-- are involved in computing where the animal is in order to get a particular disturbance in the electric field. It's not an easy task. And it's a huge computational problem, and there are computational neuroscientists still trying to figure that out.
All right. No placental mammals have electoreceptor abilities. But one non-placental mammal does have such an ability. Do you know which one? It is in the chapter. Sorry? The duck-billed platypus, yes.
How are this animal's electroreceptor inputs different for electrosensory fish? They use a different cranial nerve. And somebody said it. Anybody else know? I think you said it before. It's a trigeminal nerve, that's right. But not in those fish-- just in the duck-billed platypus. It's in their bill.
So how do they feed? They stick that bill down in the muck and in the water. And they're detecting electric fields. Many animals do emit some kind of electric fields. I don't know the details for the duck-billed platypus. I know a little more about the fish.
Another sense that is not highly developed in mammals is infrared detection. In what animals is this particularly important, and what cranial nerve is involved? Anybody else? Somebody that's not talked today yet.
The pit vipers, yes. Snakes. But only particular snakes. Snakes, like the rattlesnake. So here, in this little picture-- I think this is the same one I used in the book-- I added to the picture I found in a drawing in the [INAUDIBLE] book.
But this is the pit. It literally is a pit. It's recessed. What would be the value of that? Why put the infrared detectors at the bottom of a little pit in their face?
AUDIENCE: [INAUDIBLE].
PROFESSOR: Yeah, because you just imagine, I have a cup here, and the receptors are back here. Now it's got directionality. Now it's not responding to things over there. It's only responding to things in this direction.
So they have to move their head, of course. It doesn't give them directional selectivity if they keep their head still, except they know it's right in front of them. And you can see, it comes in through a branch of the trigeminal nerve to a particular group of secondary sensory cells and then through a circuit involving the trigeminal neurons.
It goes from there to the optic tectum. Remember, we call it optic tectum, but it actually gets many different sensory modalities-- distance receptors, it gets auditory somatosensory, and visual. And now, as we see, it gets information in these snakes from their infared detection. So they can use it to orient towards their prey.
AUDIENCE: [INAUDIBLE].
PROFESSOR: No. Only the pit vipers have that. And it would be interesting to look to see what other kinds of infrared detection you have in animals. I'm sure other animals have evolved that. It's too useful to evolve only in one small group like that.
Bats are not the only mammal with echolocation ability, another special sense. So you'd expect specializations in the brain of the bat. But what other mammals have echo reception ability?
AUDIENCE: The dolphin.
PROFESSOR: The dolphin, OK. And what cranial nerve do you think is expanded? I've actually studied that with Eric Montie, who's a marine mammalogist. Using MRIs, we were able to reconstruct the brain of the Atlantic white-sided dolphin.
And it was confusing at the beginning, because I had never seen-- I mean, I was helping him identify everything. And I thought it must be the trigeminal nerve. Any mammal I've ever seen, the trigeminal nerve is the biggest, but not in that animal. It was the eighth nerve, the auditory nerve.
And similarly, if you look at the surface of the midbrain, look at this enormous inferior colliculus. The superior colliculus is relatively smaller, and it's the same in the bat-- at least very similar. The dolphin, of course, has an enormous endbrain too, and so I've taken a picture there with an endbrain that's been removed in the dolphin, but not the bat.
AUDIENCE: [INAUDIBLE].
PROFESSOR: Humans that use some echolocation, it does become specialized. And there are indications that blind people, that are more likely to be able to use such an ability, do have changes in their cortex. And we're going to talk a little bit about that towards the end of the class. It's a very good question.
Just notice here, the other two animals in this picture, it shows animals where the superior colliculus, the more anterior surface of the midbrain, is enlarged. Are they're very visual animals.
Here's a goat, a wild goat, an ibex. And tarsier, a prosimian primate. It can literally locate using that structure. It's got this innate ability to orient and reach out with his hand and grab insects literally right out of the air.
All right, what is a brain manifestation of the specialization of primates for vision? That includes us. Most of the primates have very special-- they're very specialized for vision. That's why monkeys are used so much in studies of vision, because of their similarity to humans. You know, rats used to-- and cats used to be the most popular animal, but no longer. It's because of the specialization.
So you see, what has expanded so much? It isn't that tectum. It's not like in the ibex and the tariser here. But it's a part of the brain more involved in visual learning. And this shows an owl monkey. There's the striate cortex, what we call primary visual cortex.
But every area that you see outlined with the black line, and many of them are also in different colors, are separate representations of the visual field or separate in some way in the electrophysiological recording studies. They all have at least a partial representation, separate from the others, of the visual field. So you can see how the visual areas have expanded into the posterior parietal region and the temporal region-- from the middle temporal area here, all the way down into the inferior temporal lobe.
What's the specialization of small rodents for using their whiskers? What's happened in the brain of these animals? They have a specialized sense too, but it's not like any of the others we just looked at it, and we don't have it.
The barrel fields, each barrel-- named because of the way it looks in histology-- represents one whisker. And these are sections of the cortex that have been-- they try to flatten the cortex and cut it, or at least they cut it tangential to the surface. And here they've taken different levels, and they go down through this area of the parietal lobe, and they see these barrels. We'd have to blow that up to see how these really look like barrels. They're a crowd of neurons, packed neurons around the edges, very few in the middle.
And if you look in the middle of the barrels, all the cells would be in the dark areas here. This is stained for axons. You see, the axons from the thalamus come up representing each whisker coming up in the middle of those barrels and terminating. So it's a very specialized organization. It's been very useful for electrophysiological studies.
Other somatosensory specializations exist too, like the hand with apes and raccoons and certainly humans, because we have such high acuity and motor control and also expanded cerebellum, [? for ?] coordinating those movements.
And I mention here the prehensile tail in the spider monkey. And you get expanded representation of that tail in sensory and motor neocortex. And this just shows the raccoon. This is a picture from the book. And it's comparing the coatimundi and the raccoon. They differ tremendously in their use of their forepaw.
The raccoon literally has a sensitive hand, just like we do. And if you look in the cortex, this whole area outlined in red there-- here's an enlargement of part of it-- there's a separate little gyrus for each digit. They have very fine sensory ability and corresponding motor control of that hand.
Whereas if you look at the coatimundi, who also uses her forepaws as many animals do in feeding, the corresponding area is much smaller. And he doesn't have a separate gyrus for each digit.
AUDIENCE: [INAUDIBLE].
PROFESSOR: He does have enhanced sensory abilities, yeah. And what may be a brain specialization of the human ability for complex social interactions? And this would apply to any other primates that live in groups with complex social behavior. What do we think? There's an increasing amount of work on this now, especially the people studying the social brain here in building. Yes?
AUDIENCE: [INAUDIBLE].
PROFESSOR: In the neocortex, especially prefrontal area, which is frontal lobe association areas. We'll be defining all that later. These are the areas that keep localizing higher functions to. And we'll talk about their evolution.
And I just want to point out, I want to ask you if you remember, what the specialization that I illustrate in the book, in the echidna, which is the spiny anteater of Australia. He's got an enormous prefrontal cortex. Why would he have that?
AUDIENCE: [INAUDIBLE].
PROFESSOR: You think it has to do with it? This is the pink area here. It's relatively larger even than in humans, just in relative terms. And the colors here are the motor area, somatosensory area, visual area in green, and the darker green is the auditory area. And we would call them association areas.
But look at that prefrontal area. So I assume it must have to do with the specialized feeding. He's an anteater. And ants are very small. Obviously maximize his intake. I think there's tremendous demand on his working memory. In a single glance, he has to remember where dozens of ants are, where they're disappearing, and he's got to be able to go after them.
But that has never been analyzed. One way to get it to be analyzed is to put it in a book and raise the issue so people will look at it.
This is a question for you. What other specializations might have noticeable brain manifestations? I want you to think of something that I didn't mention in the chapter. What other specializations in animals that you know about must have some special brain representation? Sorry?
AUDIENCE: [INAUDIBLE].
PROFESSOR: Anybody? Anybody else?
AUDIENCE: [INAUDIBLE].
PROFESSOR: OK, that's never been investigated. Do they have a specialized vestibular system? What would you expect in that case? Anybody else? Specializations? Yeah. Go ahead, Rachel.
AUDIENCE: [INAUDIBLE].
PROFESSOR: Parietal neocortex, parietal association-- the area where we retain long-term memories, the map of the environment. That's right, there is some evidence for that. It's a correlation, and it's difficult to prove cause and effect, but it's a very interesting correlation. These are London taxi drivers.
We know that the cortex is affected by learning in pretty interesting ways, some of which are still being discovered. I asked my wife this question this morning, and she came up with specializations in the nose of some animals. What was the one you thought of?
AUDIENCE: [INAUDIBLE].
PROFESSOR: The star-nosed mole. John Kaas actually turns out to be one of the guys who's wrote an endorsement of my book, so I like John Kaas. And he has studied the star-nosed mole and published a representation of that.
It looks almost like a hand, multiple fingers. It's on their nose, and they use it when they go through the ground. And they use it for feeding. Yes?
AUDIENCE: [INAUDIBLE].
PROFESSOR: That's another thing that we'll be dealing with a little bit, but you're right. There are changes that occur in blind people. And that's been studied in animals in very interesting ways. And I would just add the one other animal with a specialized nose, the elephant. He's got incredible motor and sensory ability in that nose, in that trunk.
All right. This is the bigger class. We won't quite get through it today, but we'll do our best. I want to give you an overview of forebrain structures and introduce the neocortex.
I want to start with this question. What can an animal do without a forebrain? And this has actually been done. It's a pretty gross lesion. They either disconnect the forebrain from the midbrain with a cut. In some cases, they leave some of the connections.
So the initial studies were done in the cat, but then they were done on rats and pigeons. It's all in early literature. And let me just go through some of that. You call that a decerebration. Basically we move the cerebral hemispheres or completely disconnect them from other parts of the brain.
So this is our earlier diagram. And what I'm showing here is the division-- spinal cord, hindbrain, midbrain. And everything from here on is the forebrain. But the cerebral hemispheres are everything in front of this line here. This is thalamus and hypothalamus. They're tweenbrain.
So they're disconnected-- all of these structures. And of course, in the mammals if they're doing it, this area has expanded tremendously. The neocortex has grown. So they get rid of all of that.
Why do you think they've got huge differences between the results in the cat and in the rat? They both are mammals, they both have a neocortex, they both have a corpus striatum. And in both animals, they disconnected those areas from the lower brain stem, or from the brain stem period, from the entire brains.
They got big differences. What is the main thing I claim in the book is the difference? Because the early interpretations were not this. And there's been all kinds of arguments about it. Yes?
AUDIENCE: [INAUDIBLE].
PROFESSOR: Yeah, the quantity-- the number of connections severed. Very good. Why would you be disconnecting so many more connections if you're dealing with a cat than in a rat? They have the same kinds of connections in qualitative terms.
But think of the size of the cat hemispheres. He's got the highly folded cortex. He's got a relatively large neocortex, whereas the rat's a smooth-brained mammal-- in relative terms, a much smaller cortex.
AUDIENCE: Does this mean that it would cause more damage in the rat?
PROFESSOR: Well, that's what we're going to consider. Why? Why is that? I claim it's quantitative. Let's go through this. But there's another question here. Actually, both rats and cats, the only way you can keep them alive after this kind of lesion is to force feed them. Why was that the case?
They couldn't keep themselves alive. Does that mean they weren't hungry? Well, in a sense, that's true. They never showed signs of hunger. But if you put food in their mouth, they ate. So it's not true that they wouldn't eat. They just didn't show the signs of hunger motivation.
Now, the extensive study of decerebration in the cat was done by these guys, Bard and Macht, published in '58. They called it the animal a purely reflex animal. All he had was the reflexes, they say. He couldn't do any act that requires a series of reflexes, that's the way they put it. They were still very affected by the SR model of behavior. And they thought, they just couldn't put the reflexes together. We would say it more broadly now in terms of sensory motor responses.
So let's look at what the animal can actually do, and can it learn anything? First of all, the cats were anosmic and blind, because those are two forebrain senses. The cranial nerves for olfaction and vision come into the forebrain. They are disconnecting the forebrain, so of course, vision and olfaction can affect their behavior.
They didn't eat spontaneously, as we said. But they also didn't groom themselves. They showed no spontaneous sexual or other social behavior. They could, though, illicit sexual reflexes by stimulating the genitals. They could get lordosis response from females. They could get penile erection. But it required stimulation. They didn't show it in normal behavior.
OK, let's look at what they could do. They could stand. They could sit. They could right themselves if they tipped over. They could walk. They did show some abnormalities. They even showed rage, but only if you pinched them, especially their tail.
They didn't bite or strike out normally. They showed some autonomic regulation. They showed piloerection. They would fluff up their hair in response to cold. They had some thermal regulation. It wasn't as good as normal. Their temperature would fluctuate more with changing temperatures of the environment, but they still had some thermal regulation.
So commonly, if they wanted to have an easier time maintaining these animals and not have to give them injections of electrolytes and things, they left the hypothalamus attached to the pituitary, even though it was disconnected from the midbrain. They left that hypothalamic island attached to the pituitary, and then they had much better regulation of their internal environment. They couldn't learn much. They had conditioned eye blink, condition respiratory changes. But they didn't maintain it very long.
So what was it? I just said this-- describe the behavior that shows how a cat without a forebrain shows no hunger and yet will eat when you put food in its mouth. So what's he doing? He's responding to the simulation. He will open his mouth, touch his lip. He will close his mouth on the food. What does that if he's not hungry? Sorry?
AUDIENCE: [INAUDIBLE].
PROFESSOR: No, I wouldn't say it's procedural memory. I would say it's innate reflexes. Exactly, like the baby suckling. Except babies, the suckling will vary with how hungry they are. Here, it didn't seem to. But that's the question. Maybe it did vary a little bit with how hungry they were. But the point is, they didn't show any motivation.
AUDIENCE: [INAUDIBLE].
PROFESSOR: That's a big issue in how the dopamine pathways, the role they play in feeding. The representation of the motivational state, the drive state, there's no longer-- it doesn't mean they don't have it if they left the forebrain there but just disconnected it, but it means it can't influence the midbrain, hindbrain, and spinal cord anymore.
All right. So then this just summarises if you do the same thing to a rat, he can do more. He's faster to recover his righting reflexes and locomotion. I said the cat had righting reflexes and locomotion, but in fact he lost it for a while. It took him a long time to recover it. The rats recover faster.
They did show some eating and drinking responses. But again-- and more than the cat did. It was a little more than the normal, just simple reflexes. But they didn't seek food, so it was still fatal if they didn't force feed them.
And now the rats would groom themselves. The cats never did. So against that Bard and Macht conclusion, they did show a series of-- you could call it a series of reflexes if that's the language you want to use. We would describe it as a fixed action pattern, inherited pattern of behavior.
They also showed some defensive behavior that went beyond what the cat did. They would localize, they would try to escape. They would claw and bite. They even showed some auditory localization in space. Remember, the audition is the eighth cranial nerve, and it comes into the hindbrain. And it can reach the midbrain and cause orienting movements.
Why did the rat do that and not the cat? So some people said, the cat's forebrain has taken over more of the function than the rat forebrain. But as the upper parts of the brain involved, especially the cortex, it takes over lower functions.
That was the way people thought about it. And I'm asking, is that the best way to describe the results? And I say, no. So some people said, well, you take the cerebral hemispheres away, they lose all their learned behavior.
Let's look at that by looking now at another animal where decerebration has occurred, and that was in the pigeon. These were earlier than the other studies, earlier than the cat and rat studies. They were done by Dutch people, and it was all written in German-- Visser and Radamaker. And in 1935 and '37, they published this.
They showed after decerebration, they showed their basic repertoire of unlearned reactions. They would fly if you threw them into the air. But they wouldn't start flying spontaneously. You had to throw them into the air.
And while they were flying, they would avoid vertical sticks. Of course, this was all done indoors. They would land on horizontal sticks. But then they found out they would land even on the back of a dog or a cat. So what's happened? They forgot dogs and cats can be dangerous to pigeons.
There was a lot of indication that more complicated behavior patterns that depended some degree on learning during development were lost in these animals. So my hypothesis is that the forebrain is very important in linking together by learning the species' typical action patterns, fixed action patterns that are built in, and therefore genetically determined. But we still learn to link those things together.
We inherit the ability to do this and to do this, but that doesn't mean we learn how to grasp a corner. That doesn't mean we learn how to play the piano. It's not innate. Just the movements are, but we link them in different ways by learning. And that's mostly a function of the corpus striatum.
But first I want you to understand Nauta's terms. He uses these terms-- stability of the internal environment, stability in space, and stability in time. And they involve different parts of the brain. So without a forebrain, Nauta said, they still had pretty good stability in space, meaning they could balance, they could right themselves, they could move around. They had quite a bit of stability in the internal environment, but that was much better if the hypothalamus was left intact.
But their stability in time was really messed up, because their behavior is just-- their actions seemed to depend on the current inputs. And they had little or no motivation initiated behavior, little or no long-term memory. And of course, when you're dealing with mammals, they lose a lot of sensory and motor acuity. They don't have fine movements. They can't respond to detail the way they can with the cortex.
If you spare the corpus striatum, they could do a lot more. I'm not going to spend a lot of time on that. You can read this. It's all in the chapter. They've also varied-- instead of taking out the whole forebrain, they just do decortications in various animals. And the basic conclusion of those studies is that the more of the cortex you take out, the more deficient the animal is in its sensory motor control.
Again, a lot depends on quantity. As much as people tried to explain the behavior, the effects of these lesions in terms of specific cortical areas removed-- and there was some correlation, but it seemed that the biggest factor was just the amount of cortex they took out.
So that leads us to explaining this term invented by Von Monikow a long time ago when he discussed corticospinal diaschisis. It's a very interesting analysis. What is meant by that term? What does it mean, diaschisis?
You know what a schism is-- cut something into two parts. Diaschisis, separation of two things. So I want to know why is understanding of this phenomenon so important in interpreting these species' differences in brain lesion effects, and in fact in interpreting recovery of function in the human beings with brain damage. And that's why I think it's so important to understand it.
In simple terms, it means deafferentation depression. If you deafferent a structure, it means you remove the inputs, or at least a lot of them. It's always a partial deafferentation. But with greater deafferentation, the greater the effect on those neurons.
So for example, take the phenomenon of spinal shock. Do you know that term? Have you ever heard that? Has anybody ever heard of spinal shock before? What is it?
AUDIENCE: [INAUDIBLE].
PROFESSOR: But here, you're thinking of deafferentation as removal of sensory input. And you're right, that will cause deafferentation of the dorsal horn neurons that are getting those inputs.
Now let's talk about spinal shock. It's a lesion of the spinal cord. If I get a spinal cord transection-- say, at the bottom of the cervical region-- I don't just lose control of my legs and much of the control of my arms. No, I lose much more. I lose even spinal reflexes. I don't even show withdrawal reflexes or stretch reflexes. The reflexes go.
But wait a minute, they're spinal reflexes. That's called spinal shock. It takes a long time for spinal functions, simple spinal reflexes that don't involve pathways outside the spinal cord, to recover.
So here's my diagram of it. And here I've taken two species. I'm showing something like a cortex here and an animal with a lot of it and an animal with less of it. And here's a little diagram of the spinal cord, and I'm showing a reflex pathway. I just show neurons coming in and other neurons going out, and they're connected in some way [INAUDIBLE].
OK, and then you do this. You eliminate all those corticospinal connections. This is really a simple way to depict what Von Monikow described when he first described diaschisis and he talked about corticospinal diaschisis. I just put into diagrams what he was saying.
I'm showing excitatory connections. We'll ignore the inhibitory ones for now. A lot more excitatory connections removed from the spinal cord in the animal with the larger cortex.
So what happens? All these fibers are degenerated. These neurons are less deafferented in the smaller-brained animals, tan these. That means the diaschisis effect, the degree of deafferentation, is greater in the larger brained animal.
So the spinal cord reflexes, the spinal reflexes, will take much longer to recover. If I transect the spinal cord of a frog, in not many days, his spinal reflexes will recover. If I do it in a dog, it might take weeks. And if I do it in humans, it might take months, just to get the spinal reflexes back.
Why? Because the neuron can fire action potentials only if there is a depolarization of the membrane at the axon hillock that reaches a critical level. You know that from basic physiology.
If you remove a lot of excitatory inputs, then there will be more inhibitory inputs from spinal interneurons that are left, and you just can't bring the cell to threshold anymore.
OK, so then what happens after such lesions? These two things happen. There's collateral sprouting in the cord, collateral sprouting by remaining inputs. There's also what we call denervation supersensitivity. That means the neuron adjusts by increasing the number of receptors that respond to the neurotransmitters of the axons that are now missing.
So it will respond more strongly to other inputs that use the excitatory neurotransmitter. OK, so this animal will show some sprouting here. This animal, because he has more deafferentation, will show more sprouting, and there will be more receptors on these neurons, and it will be more on the animal with the larger cortex. You'll get more supersensitivity. Those are the two factors.
And just over the time course of collateral sprouting, what we know about and that has been studied, you get quite a bit of recovery from this deafferentation. So basically these quantitative effects have to be considered. Whenever I get a brain lesion-- hopefully I won't, but if I did-- if I get a brain lesion, I can't just consider, well, what were the functions now removed? I've got to consider the areas that that area was connected to and how much deafferentation that it's caused. I will lose midbrain function too, if it projects heavily to the midbrain. That's been studied-- corticotectal diaschisis.
All right, what part of the forebrain is most involved in the changes that occur during habit formation? We call it procedural learning or implicit learning. Anybody? It's the corpus striatum. And we'll be talking about that. We already mentioned it. Remember, I said early inputs, very early, when olfaction was the main sense, before there were anything except olfaction coming into the forebrain or the endbrain, it was all olfactory. If they went to the striatum, that was the link to the lower structures that control movement. And those connections were plastic. They could be changed depending on the results, the movement.
All right, so what about the tweenbrain? Just think of the main inputs to the tweenbrain. And it's relevant to this question. According to the suggestions in the chapter, what is the reason why sensory pathways ascending to the forebrain almost always have a connection to the thalamus? Even the olfactory, actually, to get to the neocortex, have to go through the tweenbrain. But why would that be? Why don't they just go directly?
So let's look at the early inputs. Optic inputs were very early. And this is the picture of it. Here I'm showing them coming into the pineal area, the pineal eye. And here's a lateral eye. The most primitive connection there goes directly to hypothalamus.
What is it doing? It's controlling the activity rhythm, the changes with the day-night cycle, the cycle of the sun, in just about all animals. That is a system that's modulating the entire central nervous system. That's what sleep and waking is-- it's a cycle of change that affects the whole nervous system.
There's many other system-wide modulations too. The pituitary here, another area where secretions affect the whole animal, the whole nervous system. And there's more than that. Hypothalamus is affecting what goes through the thalamus. It's a modulator. There are connections from hypothalamus to the thalamus. We'll go over that in more detail later.
And I think it was that modulation of the whole system that made-- it was important enough that I think it prevented the evolution of more direct connections. There are a few more direct connections that have evolved, but very few. Almost all the sensory pathways that go to the neocortex stop in the thalamus.
OK, another part in those decerebrated animals that you're removing is what we call the limbic endbrain-- basically all the parts that are connected to one structure. You know what I'm talking about? The limbic system is characterized by close interconnections with one portion of the upper brain stem. What is it?
You can define the limbic system, those structures at the fringe of our hemispheres-- limbic means fringe-- they're the more primitive parts that were there before any neocortex evolved and expanded. What was the structure? You didn't get that? Sorry?
AUDIENCE: [INAUDIBLE].
What was it? Not the neocortex, because the limbic system is more primitive-- it was there before there was a neocortex. It's this structure here. And I'm showing here hypothalamus and how it is interconnected, not only with the olfactory system but with all these structures of the older parts of the brain, the whole ancient pallium. We call them the dorsal pallium, the medial pallium and dorsal pallium up here, the medial pallium is the hippocampus, and then the lateral pallium, which is olfactory cortex, and the ventral pallium, which also gets olfactory input, includes the amygdala area.
Those areas are all interconnected with the hypothalamus. And that pretty much defines the limbic system. And there are structures also in the midbrain that Nauta pointed out should be part of that because they are also closely interconnected with the hypothalamus and with the limbic system [INAUDIBLE].
All right, I want you to read the rest of these. I'll redo a little bit of it at the beginning of the next class. But I will mark this, this is where we stopped. And then I will post these online. And we'll be able to finish this quickly next time and go on to class seven.