Flash and JavaScript are required for this feature.
Download the track from iTunes U or the Internet Archive.
Description: This lecture focuses on forebrain evolution, though it begins with a summary of auditory specializations.
Instructor: Gerard E. Schneider
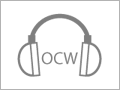
Lec 26: Forebrain evolution
The following content is provided under a Creative Commons license. Your support will help MIT OpenCourseWare continue to offer high quality educational resources for free. To make a donation or view additional materials from hundreds of MIT courses, visit MIT OpenCourseWare at ocw.mit.edu.
PROFESSOR: I want these auditory system specializations. I'd like to say just a little bit about that. Earlier in chapter six, we talked about the echolocating animals-- the bats and dolphins-- and we pointed out then that they have this very large arbitrary midbrain, the inferior colliculus. In most animals-- the rodents we look at in the lab, any of the primates-- superior colliculus is much larger than the interior colliculus. Here's one primate-- the tarsier-- and here's an ungulate-- the ibex. And you see the superior colliculus being much larger, which is typical for an animal dominated by vision. Even if they don't have a huge visual cortical area, they will still have a large midbrain area for vision.
But the echolocating animals have a much larger inferior colliculus because some of the processing is done cortically. And the inferior colliculus is the major route to the auditory thalamus.
This is from an early study of a bat that uses echolocation. And there is a lot of cortex devoted to auditory processing. And early on, they knew the primary area was very large. They could map out different frequency representation. And there were areas like, at this point, they [INAUDIBLE] worked out three different areas where the units were responsive to various kinds of frequency modulation. And then he continued that work, and he found areas or units sensitive to range-- just how far away the animals were-- and to velocities. So they could detect a number of features of their prey that they were chasing.
Now if we go to birds-- I'm asking here what is the area in bird that's comparable to the auditory neocortex of a mammal? Does a bird have a neocortex?
AUDIENCE: [INAUDIBLE]
PROFESSOR: Sorry?
AUDIENCE: Pallium.
PROFESSOR: What's the word?
AUDIENCE: Pallium?
PROFESSOR: They have areas in the thalamus that receive auditory input, just like they have areas there that receive visual input. Where do those areas project? They do have a kind of visual cortex too. But the area-- their geniculate body usually isn't called geniculate body, remember, but it's called other things-- but it does project to a cortex-like area that is called the hyperpallium. Pallium, of course, is just another name for a cortical region. And the hyperpallium is the most similar to the neocortex of mammals.
But then, they have other nuclear in the thalamus that get input like the LP in mammals or the medial geniculate body. They get input from the midbrain. So in the case of the auditory system, they're getting projections from the bird's inferior colliculus region-- often, again, with a different name. In the birds, it's usually called nucleus ovoidalis. And that projects to this large region that for many years was called this neostriatum in birds. But now we know first from connection data gathered here at MIT by Karten, who was working with Nauta. And later, in the gene expression data, we know that that area is not equivalent, not homologous to the striatum of mammals.
So it's called a nested pallium, or a neopallium. It is subcortical if you go by the ventricle. Here's where the ventricle is along here. And the hyperpallium is above it. And that would be where the equivalent of the lateral geniculate projects for the visual system. And a lot of it is visual. And the medial part is hippocampal. But the auditory region of the thalamus, nucleus ovadulus projects to the neopallium, the medial part in an area we call Field L.
Now reptiles have something similar. We get the thalamic nucleus that gets auditory input a different name-- the medial reunions. It goes to that large dorsal ventricular ridge. And dorsal ventricular ridge becomes a neopallium if it's in bird development. We keep calling it dorsal ventricular ridge in the reptiles. And they have a auditory region, just like here more laterally in this region right there it gets visual input from a different nucleus in the thalamus, a nucleus that gets input from the tectum. And then, of course, here's the equivalent circuit in mammals. Medial geniculate body to these auditory critical regions.
And this is what it looks like. This is from an atlas by Karten working with Bill Hodos in the late '60s. And they published this atlas showing the appearance of sections at various levels of the bird brain. And you can see just looking at the cells here, how Field L stands out. It's got several layers-- just a little layer down here, the main layer there, and another, more superficial layer. And here's the way it's diagrammed. And you can see that's all below this end-brain ventricle. And this would be where the hippocampal equivalent is, the medial pallium, and this whole region in many animals is just called dorsal cortex. But in birds, it's called the hyperpallium.
All right. So if you trace the auditory pathway from the cochlear region, they don't have the coiled cochlea of mammals, but it's, instead of a basilar membrane, they have a basilar papilla. And where the auditory receptors are in contact with the eighth nerve, and you can see the eighth nerve comes in to contact the secondary sensory cells of the cochlear nuclei, just like in mammals. We saw that when we talked about spatial location, remember, for the [INAUDIBLE]. They're dorsally located. And the neurons there project to the inferior colliculus of the bird. It's got a different name-- the MLd-- mesencephalicus lateralis pars dorsalis. OK, and that then projects to the thalamus-- the ovoid nucleus-- nucleus ovoidalis. Which projects to Field L. OK, so that's just the simplest picture of the auditory pathway.
But you can then follow things from the auditory pathway that are involved in controlling their vocalizations. And the most direct pathway goes from Field L to a so-called higher vocal center, which then projects to this nucleus robustus of the arcopallium. The arcopallium is homologous to the amygdala region of mammals. Remember the amygdala region, though, has many outputs to hypothalamus and to midbrain. And in birds, this nucleus robustus has a direct projection to the hypervisual nucleus, part of which controls the syrinx, which controls basically the voice box of the bird, controlling his vocalizations, together with breathing control. And then there's a less direct pathway that goes to an outer part of the inferior colliculus region, which then projects to the hypoglossal nucleus and controls the syrinx.
Now there's a less direct pathway that in many ways is more interesting. From Field L and the higher vocal center, it projects to the striata of the bird. Now we're talking about the real striata. That is, it's equivalent to the dorsal striatum in a mammal. And like in mammals, the striatum has an output to the thalamus. And the thalamic structure it projects to then projects back to a pallial region. It's like a Broca's area in mammals. That is, they're very similar pathways controlling speech in humans. It's part of the nested pallium.
Remember this, more caudally, the Field L, the auditory system, gets part of that nidopallium. Well, [INAUDIBLE], this nucleus, called LMAN, is also involved in vocalization. In fact, this circuit is what's involved in male birds learning the vocalization of their species. And they learn by imitation of listening to their father sing, and they develop. If they don't hear that singing, they don't develop as complex a vocal repertoire. Anyway, that nucleus projects to the robust nucleus, which gives rise to those outputs to control the vocal system of the bird, the syrinx in the throat.
All right. You learn a lot about that if you work with Michael Fee in the building. And students that work with Michael probably become pretty familiar with that circuit.
But now, I want to go through some, say a little bit about the forebrain before we become a little more specialized in covering the forebrain. We'll be talking about-- well, you'll see here. We've covered now-- the first five parts were all development and evolution of the brain explaining the basic organization of the CNS. And then, we went to specialized systems. And I began with three chapters on the motor system, then a single chapter on brain states, and then sensory systems, which should probably be called special century the because general sensories, and metasensory I didn't cover in that section. Because we talked a lot about somatosensory systems in the earlier parts of the book. So that's when we dealt with gustatory olfactory, visual, and then finally, auditory systems.
So now, forebrain origins. And then we talk about the limbic system and hypothalamus. That'll be next. And then, a couple chapters on the corpus striatum of mammals, primarily, and then finally, several chapters on the neocortex. So what was most likely the earliest part of the forebrain? You first encountered a forebrain when we talked about amphyioxus, a cephalochordate-- simplest living chordates. And remember, you had pigmented cells there that respond to light. You had secretory cells secreting. Directly into the bloodstream of the animal.
AUDIENCE: Olfactory bulb.
PROFESSOR: Sorry?
AUDIENCE: Olfactory bulb.
PROFESSOR: That's a very interesting comment because you would think olfaction would probably be the earliest input. But in fact, we're not sure there's olfaction in amphyioxus. But we do know there are those secretory cells. And we do know they're getting effects of light through that pigmented cell region. And they have cells that are like retinal ganglion cells that provide input to the brain. Now we think the most primitive part is all equivalent to diencephalon. And the gene expression studies have supported that. It's actually like diencephalon, not like end-brain. So they don't have anything like cortex. They don't appear-- nothing for sure-- of olfaction. If they do have any olfaction, there are a couple of nerves that they don't know much about. One of them could be equivalent to olfactory. But it's going into the equivalent of a tween-brain.
So that leads to this next question-- what are the cranial nerves that are attached to the forebrain? Now forebrain includes end-brain and tween-brain, remember. So I want all the cranial nerves that connect there-- now one of you've already mentioned-- number one-- olfaction, number two is what? the optic nerve-- OK-- which becomes optic tract, so it is a CNS tract, but we still call it a nerve before it gets to the chiasum. OK, so that's two of them. Those are the two that are among the twelve that we name for the human brain. But there's actually three others. OK, and here I list them.
If you don't have a forebrain, he's missing these five nerves. Nerve one and two-- but nerve one-- the olfactory nerve we could include the vomeronasal nasal nerve and the terminal nerve. Terminal nerve innervates, apparently the nasal septum. It's found in humans-- at least in most humans. There's some variability. We think it may respond to olfactory input, but even that is not certain. You'll find it in human anatomy books. The vomeronasal organ is Jacobson's organ. It's in the nasal cavity.
And animals that have this well-developed have an opening into the roof of the mouth. Ungulates have this very prominently. And they, for example, in mating, the male collects the urine from the female, brings it into his mouth to that organ in the roof of his mouth where there are receptors that respond to pheromones that change with the receptive state of the female. OK, so he can then tell from that preliminary mating action whether she's receptive, and whether it can be impregnated at that time. So it's part of the ritual they go through before mating. And the other animals still collect pheromones on their tongue-- snakes, for example. You see them flicking out their tongue. They're actually collecting substances from the air. And if that includes pheromones, they bring it to that vomeronasal organ.
Where does the vomeronasal organ project? To part of the olfactory bulb-- remember, there's a main olfactory bulb and then there's an accessory olfactory bulb. And most mammals have that. And it specializes in responding to pheromones. It can come in through the olfactory epithelium also, not just through vomeronasal organ. And then, we have the optic nerve. And then there's another nerve that brings in information from light. The epiphyseal nerve-- this is the pineal-- the nerve can activate the pineal cells by light. And we know about the pineal eye. If animals have the pineal eye on the top of their head, they have a nerve that's called the epiphyseal nerve. The epiphysis is the name for that structure.
OK, so five cranial nerves, but three of them associated with olfactory, and two of them responding to light-- OK, so if an animal doesn't have a forebrain, there's another thing he's missing. He doesn't have control of his pituitary gland. Because what controls the pituitary? Well, we're going to learn more about that after this class. We deal with hypothalamus, specifically. But we know the pituitary is connected to the hypothalamus in a very interesting way that we will discuss.
It's possible, though, to disconnect the entire forebrain as we learn in-- I think it was chapter seven. You can disconnect the entire forebrain from the midbrain . But you could leave the hypothalamus connected to the pituitary. And if you do so, the animal maintains a lot more function than if you don't. Because pituitary, to function normally, needs the hypothalamus attached. And the controls that the hypothalamus exerts on the pituitary gland need to be intact. So in chapter seven, I went through studies where there was removal or disconnection of the forebrain from the midbrain. And I said that animals are missing major components of normal behavior, even if you leave the hypothalamus attached to pituitary.
So what is missing? Anybody remember? Motivation. Motivation-- motivated behavior. So we called it appetitive behavior. So if an animal's hungry, his behavior that indicates he has an appetite for food. But you can use that term-- appetitive behaviour for other motivations too-- for example, if you're thirsting. Appetitive behaviour when an animal's thirsty, a behavior initiated by his motivational system, a hypothalamus that centers on hypothalamus will lead to locomotion and seeking of water. You can use the term, also, for mating behavior, for sexual motivations. You can use it for brood-tending behaviors if they're raising young.
But there's a lot of other things missing, of course, that depend on forebrain-- all the cortical and corpus striatum functions. And I've listed major forebrain functions-- all of which we're going to be talking more about. First of all, acuity-- animals that develop a large forebrain have much better sensory acuity and much better manipulatory variability of their limbs. But wait a minute. Doesn't animals that have a very large optic tectum have good acuity? Well, they do, but only for unlearned behaviors. Acuity for learned behaviors depends on that forebrain. And of course, humans are so dominated by learning that often we're pretty unaware of the tectal controlled behavior, although it exists in primates too.
OK, but then other things they lack that you need a forebrain for. You need an endbrain for. Lack of anticipation-- they're controlled by stimuli coming in, but they can't anticipate what's going to happen. They don't have good spatial memory. They don't learn the habits. The only learning of the midbrain and lower structures is very short-term. Many studies have shown this. What we know as long-term memory developed out of spatial memory system involving the medial pallium or hippocampus. And we know that habit learning developed very early in the olfactory system involving the striatum.
OK, so what do they retain if they've lost all this? What can they still do?
AUDIENCE: They can eat if they're-- [INAUDIBLE] They can eat if they're fed.
PROFESSOR: Yeah, they can eat if you put food in their mouth. What do you call the eating? There's a general term we use for all drives for that output site. We call it consummatory behavior. It's very easy to remember for eating because it means consuming the food. OK? But you can refer to consummatory behavior for thirst. You can refer to it for sexual behavior where the consummatory behavior is mating, and so forth. So they retain consummatory behavior. They retain-- and I've listed some of those-- they do have pain-elicited aggression.
So a cat without the forebrain-- if you cause him pain, like you pull his tail-- he will strike out. He'll unsheath his claws and strike out. But as soon as you let go, it's gone. So we call it sham rage. He acts like he's enraged, but it's not enduring. We know what we call rage in an animal outlasts the stimulus that triggers it. A human can be angry about something that happened hours before. It can be angry about something he's just thinking about. He can be angry about something-- if you hold a grudge, you could be angry for years.
OK, an animal like that retains his sexual postures and reflexes. You can stimulate an animal like that, like a female cat if she's receptive, you can stimulate the lordosis response just by touching her in the right way, depending on the animal-- they have different regions. If you give them tactile input, they will assume that receptive posture. That's part of the-- you can call it a reflex, but it's a complicated reflex. It's part of their fixed action patterns that they've inherited.
AUDIENCE: I'm just wondering, how about for fear-- a sense of fear?
PROFESSOR: Like what kind of fear?
AUDIENCE: Do they still retain the fear?
PROFESSOR: They don't remember long-term. They don't retain the fear, and it doesn't last very long. It's all pretty short-term, very dependent on the stimulus. Like a pigeon will actually fly without a forebrain if you've left his optic nerve attached to the tectum Because all the stimuli are there. If you throw him into the air, all the stimuli that elicit flying are there. And he will flap his wings and be able to stay in the air. He will locomote. He will fly and he will actually avoid running into things. He will go around trees. He will land on horizontally placed things, land on a surface, land on a limb. He won't maintain the motivation, though. So once he lands, he won't have the incentive to take off again unless if he gets blown off the limb, then he will start flying again. And they will right themselves. If you tip them over, they will walk. But generally, they don't initiate much walking. They have to be nudged.
So let's talk about primitive forebrains. We can look at the nonvertebrate chordates. The invertebrate chordates. That includes amphioxus. We could look at the proto mammals. But to do that-- the animals that led to mammals-- we'd have to use paleontology and look at skulls. And that's been done. We talked about that a little bit when we talked about auditory system. Because it's through those studies that we know about changes in the middle earbones and the bones around the eye which became reduced in the earliest mammals. And of course, we can use comparative anatomy to study the brain. That is studies of living animals. We can't do comparative anatomy very much with animals. Well, we only have the fossils because brain is retained in the fossils. And of course, we can study primitive mammals.
Now we use cladistics to portray these different animals' groups. And this is a typical cladogram of the vertebrates drawn by R. Glenn Northcutt. And Glenn Northcutt has done a lot of different work in comparative neuroanatomoy. He's a little bit different from Karten in that he's sort of a systematizer, whereas Karten is a guy who studies connections and is interested in what led to what in evolution. I'm a little more like Karten. Karten was also a student of Nauda. But Northcutt and his students have done a lot of contribution to cladistics. In fact, Northcutt was probably the first neuroanatomist to use this way of portraying comparing animals.
What he's showing here is how certain animals branched off from the main vertebrate tree. This is a chordate line action-- like the sea lamprey. Amphibians-- well, then the fish, and very early, the Elasmobranches, the cartilaginous fish branched off. That includes the sharks, skates, and the rays-- and then of course, later the bony fish. So if you're at the end of an era, he shows a brain of a living animal here, sort of where that line of evolution has led to. And here the bony vertebrate-- these are all bony vertebrates-- amphibians branching off the earliest, and then the Sauropsids, reptiles and birds. But even before they branched off that line, we see the mammals.
OK, here's an earlier view. This was Ludwig Edinger, 1908. And he had a view that can easily be fitted to the cladogram, cladistic approach. But he didn't use it. We didn't have as many sources of data about evolution then. So he basically talked about this part that he saw similar to the neocortex of humans, much smaller in small mammals like the rabbit. What he doesn't show here is something major. What is the major difference in that end-brain of cartilaginous fish and lizards when compared with rabbits and humans? The neocortex-- and that's something that Northcutt stresses.
But what he shows here is the cerebral hemisphere, which again we know is different in mammals than in all these other animals. Because only the mammals have the neocortex. But if we take a cladogram here and look at the creatures that are still living today that branched off the earliest, we find these three-- amphyioxus, sea lamprey, and hagfishes. And we looked at these once before. And you'll just notice very tiny telan cephalon in these animals-- non-existent, really, in amphyioxus. They just have a diencephalon-like structure. Very tiny, smaller than the olfactory bulbs in the sea lamprey-- and still very small in the hagfish. Here's a hagfish. There's the telan cephalon. There's the olfactory bulb. The whole telan cephalon is not that much bigger than the olfactory bulb.
Then I show you here some of the bony fish-- the mormyrus with the huge cerebellum, a trout with a huge midbrain-- predatory fish, very tiny telencephalon. Here's a [? vicar ?], which is only slightly larger telencephalon.
And here's a question that you don't see very much, but Georg Striedter treats it, and so does [INAUDIBLE] in his book. They say in most chordate groups, brain size increases in evolution, but in a few groups it decreases. So why would it ever decrease? For it to decrease in evolution, that means there had to be adaptive reasons why it would decrease in size. Can you think of an adaptive reason why a brain structure would decrease in size?
AUDIENCE: Well one idea is just to make your head lighter. And this is actually going way back, but when I think of dinosaurs, sauropods had very long necks and that's just hard to support a heavy head. So they had very small brains.
PROFESSOR: So even the weight could be a disadvantage. I would say one thing is even more important. And that brain tissue-- very demanding in energy consumption. Like our brain is hardly 20% of our body weight, but it uses 20% of the energy. Some people get a fever if they think too hard. I'm sorry-- couldn't resist that. It actually uses a lot of energy. You can't go without eating too long if you're working hard on homework problems. So it can decrease because of energy demands.
Why is that so critical? With high energy demands, you've got to spend more time getting food. And it takes a lot of energy, depending on what you're eating, of course. You might be eating a high-energy food, like eating other animals. But then it takes energy to digest. So that's another big energy consumer. Brain, gut. What did humans develop that made possible the much larger brain using a lot more energy? How did humans decrease the demand on their digestive system?
AUDIENCE: Cooking.
PROFESSOR: Cooking-- exactly. So you see after the indications of fire being developed by humans, the last big surge of growth of the end-brain. OK?
AUDIENCE: How does cooking help?
PROFESSOR: I can't--
AUDIENCE: How does cooking help? Because I thought it actually took some of the--
PROFESSOR: It requires a lot more energy to digest raw meat than it does cooked meat. The energy alters the proteins in ways that assist their digestion. This is dealt with in a little more detail in Allman, but you can find discussions of it too online.
AUDIENCE: Can I ask something? Can we say that the size of a brain means that it's more smart, or something?
PROFESSOR: OK, you have to add to that that if brain size is decreasing, they must decrease the size of something that's not critical to their survival. You're quite right. We've talked about how the size of a structure increases if there's a lot of neural processing going on. He needs it for his hunting behavior or other things he's doing. You're right. Let's look at which animals-- these were brain versus body weights of the major animal groups, just to remind you where humans are. Remember it's relative size that increases-- this is whale up here. This is elephant. They have the largest brains.
Humans are here-- smaller brains, but in relative to their body weight, it's the largest. And this is on a cladogram. Striedter drew this after figuring out where the increases in relative brain size occur, where the decreases occur. There's not decreases very many places. But you see here when salamanders evolved, there were decreases in relative size. The eels-- similar. There were decreases in size. All these others-- the red dots are for when there were marked increases in size.
And remember, we talked about the very early forebrain and evidence that is dominated by olfaction. I emphasized that early in the book. And I talked about how olfaction dominated the primitive endbrain. We didn't have really good information on that until people fairly recently studied the olfactory bulb projections in those two animals that are members of the non-bony vertebrates. And that was the hagfishes and the sea lampreys. Probably the lamprey study is the most interesting because the lampreys are a little less specialized than the hagfish. This is a hagfish-- the Pacific hagfish. They injected tracers into the olfactory bulb here.
This is BO-- bulbus olfactorious, sorry. A lot of times they like to use the Latin or Greek names, so they use it here for telencephelon, then diencephalon. And what is TM? Tectum mesencephali. So midbrain tectum-- and this is just a summary of olfactory bulb projections in the hagfish and lamprey when compared to amphibians, also the most primitive group of tetrapods. Notice that in the hagfish and also in the lamprey, the olfactory bulb projects to every end-brain structure that's there and some of the tween-brain structures. This is a projection to the tween brain. And some of these structures are tween brain. This is actually striatum here. In the lamprey, it goes into the striatum. It goes into the medial pallium here. This is a layered structure. It's going into one layer and other tween structures and diencephalic structures.
But when you get to amphibians, you see it's going to the medial pallium, but only one edge of it. It's going into the dorsal pallium, which is like a parahippocampal area in those animals. And we know it projects into the medial pallium. It gets direct olfactory input, but only at one edge. And it goes into the striatum, but not throughout the entire striatum. Big areas then that don't get olfactory bulb projections the way they do in the lamphrey and hagfish.
And it's already 4:00. I think I'd better stop here. I think we will finish this, and be able to start talking about the hypothalamus next time.