Flash and JavaScript are required for this feature.
Download the track from iTunes U or the Internet Archive.
Description: This lecture begins the discussion of specializations in the evolving CNS, including sketching the brain and basic pathways of ancestral mammals.
Instructor: Gerard E. Schneider
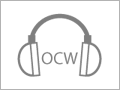
Lec 5: Specializations in t...
The following content is provided under a Creative Commons license. Your support will help MIT OpenCourseWare continue to offer high quality educational resources for free. To make a donation or view additional materials from hundreds of MIT courses, visit MIT OpenCourseWare at ocw.mit.edu.
PROFESSOR: This is now class five. We had an introduction to it last time where I talked about this question, does ontogeny recapitulate phylogeny? It's been important in the history of the field. And we know that, very roughly speaking, yes there's some correspondence but it's not perfect.
And there's probably no truly phylotypic stage for all the species in a given phylum. But nevertheless, there are a lot of similarities across species, especially when we deal with spinal cord and hindbrain, midbrain and even some aspects of the endbrain.
What is the biggest type of difference that does occur? You've got to discriminate between the topology of the brain and the detailed topography of the brain. Relative sizes of structures vary. And so after we finish this class-- I don't know if we'll get to it all today. We're going to go through some of the specializations where you will see just like we've already seen for the hindbrain, certain structures grow a lot bigger in some animals than others, depending on behavioral specializations.
OK. We found out what a cynodont was. They're the mammal-like reptiles. Mammals evolved from that group. This is an early cynodont here in the upper left. So just as a fantasy, we can pretend we're looking at the brain of an early cynodont. But it's really based more on amphibian brains and very primitive reptilian brains.
All right. So the relevance to mammals is that we still have that brain, even in the human brain. It's like it's the older parts of the brain that are still there. I already said spinal cord, hindbrain, and midbrain. You can see the same structures across many different species.
And in fact, even for the endbrain, there are great similarities. But think of that. This primitive brain, we're going to take a look at is representative of the core of our own brain. But first, let's just take the cynodont memory and you tell me what you remember from what we've learned already.
Look at this brain. I've wiped out the top part because I want you to remember. By now, we know that the most caudal part of the CNS, we have the spinal cord. And that's just an example of a spinal nerve in the dorsal root carrying sensory information to the upper part, the dorsal part of the spinal cord.
And obviously, we've distorted the brain here just to be able to show connections. So what do we have about the spinal cord? Everything between here and here. What is there?
Hindbrain. OK. What do you think that this? Cerebellum. OK. So the rostral part of the hindbrain is often called the pontine region because cells down here-- which I don't really depict in this screen, but we certainly see it in mammalian brain-- connect to the cerebellum. It carries input from the cortex. And the lower part-- the Latin for spinal cord is medulla spinalis-- the core. The medulla, the core, the inner core of the spinal column.
So the lower part of the hindbrain is just an extension of that, the medulla oblongata, the elongated spinal cord. Whereas the rostral part now is changed. That's where the cerebellum develops.
What's above that? The midbrain or mesencephalon-- well, what are the bumps up there? We see it in all of vertebrates.
Sorry?
AUDIENCE: The colliculi?
The colliculi. What does colliculi mean? It means little hills. So if you look down on the surface-- we do a brain dissection, you'll see that in the sheep brain. You'll see that in any mammalian brain. You'll see these four bumps.
And in some animals, one of those bumps, a pair of those bumps is much larger than in another species. But the basic structures are the same. OK. And then above the midbrain, if we just take this whole division-- sorry?
AUDIENCE: Tweenbrain.
PROFESSOR: Tweenbrain. or diencephalon. And the largest part up here? Thalamus. This is really crucial to our functioning.
AUDIENCE: The hypothalamus.
PROFESSOR: Hypothalamus. Below the thalamus. OK. And now above that, above the tweenbrain, we know that's all what?
AUDIENCE: [INAUDIBLE].
PROFESSOR: Forebrain. OK. The most rostral of the brain vesicles. But I've got to divided up here. This is the biggest subcortical components. This is mostly pallium up here. And in this schematic here from Streeter, he names a few more of these things.
And he explains that the pallium, the word means a cloak. What's a similar word that we use for pallium? Cortex. What does cortex mean? It means the bark, like the bark of a tree.
OK. So the pallium is the older word and we use it often in embryology. So this is the older parts of the pallium. There's no neocortex below mammals. This is a pre-mammalian brain. But that doesn't mean there's no pallium.
OK. There's our dorsal pallium, a medial pallium, a lateral pallium, and there's even a ventral pallium. But I have this down here. An olfactory structure, this ventral pallium. And then these are sub-pallial components that are connected with the pallium there.
That would include structures like the amygdala and the mammalian brain. Yes?
AUDIENCE: Can you remind me how they figured out which part is which?
PROFESSOR: How do we know which part is which? Well, that's basically what you're learning. But we know it from cytoarchitecture. We know it from fiber architecture and very important for this class, we know it from connections. OK.
We're interested in how these structures evolved and how they're interconnected and how those connections evolved. So that's what we'll be dealing with.
AUDIENCE: But these are made up of more recent amphibian brains?
PROFESSOR: That's right. But we know from the skull shapes and everything of these ancient animals that they were pretty similar. OK.
If you read on Google online and you do searches for cynodont, for example, you'll see some fairly ridiculous things. They'll say mammals are cynodont and things like this. And that they suckle their young and this and that.
We don't know that. OK. As I point out in that previous slide, just read what I had at the bottom. I summarized that.
We only have the fossil record. So soft tissue isn't preserved in the fossil record. So the brain isn't preserved. Well, neither are mammary glands or any other soft tissue. All right.
So this is meant to represent corpus striatum. This is the pallium above it. And of course, olfactory system. At least remember those three things. And remember that the pallium here is not neo-pallium or neocortex yet.
And here was the original figure and that is in the book, or a more modernized version of it. And you can see, I've labeled there the different parts of the pallium you find out there. Even the most primitive of all the vertebrates, like the sea lamprey, has a pallium. But we can only really see a dorsal pallium and the ventrical pallium.
And this is the more realistic view where I take an embryonic brain. I've shown a dorsal view, except I've taken the hemispheres and I pushed from apart. This is before there's any corpus callosum that's evolved.
So this isn't a section. OK. It's a surface view with the hemispheres pushed apart. So if you want that to look more like the embryo would look, like in 12 or 13 embryonic day hamster or mouse, take these and push them together.
The striations there represent a thin one-layer thick layer of cells. It's one cell layer thick as the roof plate. Remember how the roof plate there you see in the cross section of the hindbrain. The roof plate where the cerebellum actually forms rostrally. But in the early embryo I'm showing in here before the cerebellum is grown. And the cerebellum does develop relatively late.
And this roof plate-- there's an area of thin roof plate in the medial side the hemisphere and on the dorsal part of the tweenbrain that you see right there.
So now can anyone answer this for me? Let's talk about some of these neurons that we see in that diagram. What are three different types of primary sensory neuron structures? And then we'll talk about where we find them, even in mammals. What am I talking about?
Primary sensory neurons. Let me start at the answer just by saying some primary sensory neurons are right in the epithelium, just like in an earth worm.
Where do we have those in our brains, in our nervous system? Where is the primary sensory neuron right in the surface? The cell body right in the surface. In here, in the nasal epithelium, OK. Your receptor cells, olfactory receptor cells are the primary sensory neurons. And their dendrites are highly specialized to respond to things dissolved in the nasal mucosa. It responds to odorant molecules that we breath in.
But you don't respond to those odor molecules directly from the air. They have to dissolve and stimulate receptors in the dendritic components of those primary sensory neurons.
What are the other types?
AUDIENCE: Bipolar.
PROFESSOR: Bipolar cells. In some animals, that's the main type of neuron carrying sensory information from the body surface. OK. Where do we have those? Anybody remember? Sorry?
AUDIENCE: Vision?
PROFESSOR: Not in there. Well, that's a good-- that's actually a pretty good answer. Because they are bipolar cells. That's right. We often forget that. Because the retina is so highly specialized. OK. But yes, in the retinal cells, we have bipolar neurons. Where else?
For primary sensory neurons, no. Here, in the auditory system. And where else? Vestibular. OK.
Auditory and vestibular are bipolar. And this is the way Cajal depicts them. He's showing different animals here. Besides, it's the only one I have here. He's showing different animals. OK, but we have all these in mammals. In the epithelium, a bipolar cell sub-epithelium, and bipolar , cells where the cells are collected into the ganglia.
And then the fourth type is the major type in us and most in mammals and birds. They're called pseudo unipolar. They're unipolar in their shape but in fact, they still have a peripheral process and a central process.
As a big advantage, conduction can be faster because the axon is continuous here and this is now the metabolic support system. It has nothing to do with the action potential, OK. But it supports the cells, of course. You kill the ganglion cell on this axon will die.
So there's most of the neurons carrying input from the skin are unipolar in mammals. There's a bipolar cell. And here's the olfactory epithelial primary sensory neuron, carrying input in for the CNS.
All right. Now here's a different kind of question but still sticking to the more peripheral parts in the nervous system. What are the different types of muscle cells? Now in the chapter, I talk about just two major types.
We could come up with sub-types of these two. But what are the two main types? These? What types of these?
AUDIENCE: Striated?
PROFESSOR: Striated muscle cells. OK. And there's muscles here, too. This is a cross section of the gut. OK. That's lined with muscle cells.
What kind of cell is that? You see them in the lungs, in the diaphragm.
AUDIENCE: [INAUDIBLE].
PROFESSOR: It's called smooth muscle. Not striated muscles. And the microscopic appearance is very different. You don't have the alignment of the cells-- it forms these striations that you have on the striated muscle.
There is a major type of striated muscle cell that actually is innervated by the system that innervates the visceral. What type am I talking about? Heart cells, right. Cardiac muscle cells are striated cells. But their innervated differently.
OK. Let's see if anybody knows-- let's do seven here because that's related to what we just talked about. What are the two main types of motor neurons depicted in this introductory chapter? And you see them here. Two major types of motor neurons.
AUDIENCE: Ganglion--
PROFESSOR: Well, that's one way to call them. These are the alpha motor neurons with the axon of the motor neuron defined as we already defined it-- the axon that leaves the central nervous system. They innervate the striated muscles.
And here's another motor neuron. But it doesn't contact the muscle. It's still called the motor neuron. It leaves the central nervous system, the axon and it contacts the ganglion cell out there.
This is a neuron that's located in peripheral ganglia. And then that's the way the gut is innervated. The same is true of the heart. It's always an innervation of the ganglion first, the ganglion cell. So we call this the postganglionic axon. This is the preganglionic axon.
OK. So those are the two major types of motor neuron. There is another that we don't depict. I don't depict in these diagrams but these are cells that secrete something into the blood. They could be called motor neurons. But here, we're just calling them motor neurons because they have something to do with movement.
It's not secretion. But it is a major type of output of the central nervous system. OK. And does anybody know what secondary sensory nuclei receive input from axons of the eighth cranial nerve? They're in the diagram. The eighth cranial nerve.
This is an axon of the eighth cranial nerve. You know when you're a neuroanatomist, if someone gives you just the number of a nerve, you know what it is. The eight nerve always means what? Auditory and vestibular, OK.
So I put there a spinal nerve, the eighth cranial nerve and the first cranial nerve. That's what I show here in this diagram. Now in this diagram, I want you to remember what we said about interneurons an what I write about it. I want you to discuss the term interneuron. It has a broader meaning and a more commonly used meaning in discussions of neuron anatomy.
So a simple way-- the broader definition, how would you define it most broadly? What is an interneuron here? Yes?
AUDIENCE: Anything that's not primary sensory or primary motor.
PROFESSOR: Exactly. OK. So it's not one of these that I show black in here at the bottom. Because its axon, those axons are leaving the central nervous system. And it's not these that also have a cell body outside. Normally from the term interneuron though, we exclude the secondary sensory neurons.
Although they could be called interneurons in the very broadest sense. They're in between primary sensory and motor. But now if we also exclude the secondary sensory cell, it's everything else. But what is the more common meaning when you hear an anatomist talk about an interneuron?
AUDIENCE: A smaller--
PROFESSOR: A short--
AUDIENCE: [INAUDIBLE].
PROFESSOR: Yes, short axon is the term we use. The short axon interneurons. Sometimes we abbreviate short axon interneuron and just call them interneurons. But actually, every cell of the great intermediate network, even these with the long axons here, are all interneurons.
But sometimes, we would use the more-- we'll just be talking about short axon interneurons. The most common way for any axon in the brain to affect movement is through a short axon interneuron. That's true here in the spinal cord.
We always think of primates and we think, oh, but the motor cortex controls the motor neurons directly. That's even in primates. It's not the most common type of connection. It goes through interneurons.
We call it the interneuron pool, a pool of neurons that all innervates the motor neurons. It causes movement. Very important in talking about how the motor system is organized.
OK. Now let's talk about the local reflex channel of conduction. You can talk about different channels where information coming in, like through one of these axons, is rooted. And the first one is a local reflex channel.
So what is it in the strictest sense? A local reflex channel here? Here's the axon coming in. I'm just going to draw the shortest pathway to a motor neuron. So you could get movement now. That's a local reflex pathway.
So when we deal with spinal cord, we could define it as a limited-- where the conduction is limited to one segment of the cord. OK. So we talk about segmental reflexes.
What would be a possible function of that pathway I just drew?
AUDIENCE: That you adapt faster than you're able to have processed.
PROFESSOR: OK, but can we name the type of reflexes? One synapse here. Another synapse here. Another synapse here. What might be the function?
AUDIENCE: Pain?
PROFESSOR: Yeah, a response to pain. That's a good answer. But what kind of reflex is that? How do we respond? If I reach out here and something suddenly hurts my finger, what kind of reflex is that? It's got two names. Withdrawal reflex or flexion reflex. OK. Withdrawal or reflection reflex.
It always involves more than one synapse between the primary sensory neuron and the motor neuron. There is a reflex that only has one synapse, the mono-synaptic reflex. What kind of reflex is that?
You learn this in 901. At least when I taught 901, I always made sure everybody knew this. The axon, I actually showed you a picture of it already, from Cajal. Except he drew it the input coming from skin and I said that was wrong, it comes from muscle.
These little sensory receptors in our muscles, especially the muscles of fine movement. OK, we've got a lot of little receptors in those muscles that detect muscle stretch. The stretch receptors. They give rise to secondary-- the primary sensory neurons innervating them have very large axons, the 1a axons.
They're fast conducting and they go right to the motor neuron. A motor neuron innervates the very same muscle where it came from. And the reason it's such a nice system is that there's a little muscle inside each spindle organ.
So the sensitivity to stretch can be varied from the central nervous system by a little motor neuron in the ventral horn called the gamma efferent neuron. You're constantly adjusting those muscle spindles. So you can actually-- your brain can set the link at which the spindle starts firing. It's very nice way to get stable, control the movement. All right.
So this kind of axon that enters one segment of the cord comes from that. What does that mean? A dermatome.
AUDIENCE: It's like a map of where the sensory inputs into the spinal cord are laid out.
PROFESSOR: Yeah. It's true. But I want you to be a little more specific. How is a dermatome defined? How is the first thoracic dermatome defined? Now I'm giving you a huge hint. It's an area-- what does derm mean?
AUDIENCE: The skin.
PROFESSOR: The skin. Tome-- section. So it's got to be a section of skin. What kind of section of skin? There's a dermatome map. What do each of those little sections represent? The skin innervated by the dorsal root of one segment.
OK. Meaning, we should find eight cervical dermatomes. We should find 12 thoracic dermatomes. We should find five lumbar dermatomes, five sacral dermatomes. Some people might define one [INAUDIBLE] dermatome.
But generally, it doesn't. Usually, we just talk about down to the sacral. Why does it have such a peculiar arrangement on the limbs and such irregular arrangements on the trunk? That's one question. Another question is, what about the face? Why isn't the face included?
AUDIENCE: There's no connection to the spinal cord.
PROFESSOR: Sorry?
AUDIENCE: There's no connection to the spinal cord.
PROFESSOR: Yeah. It's not innervated by the spinal cord at all. That's right. What is it innervated by?
AUDIENCE: The cranial neuron?
PROFESSOR: By what kind of nerve? Cranial nerve. Which cranial nerve? What's another name for the fifth nerve? It's got three main branches so we call it the trigeminal nerve, exactly.
OK. Very good. Put the human in this position and now imagine he's still an embryo, and the limbs are just little stubs here. Then you will see that the lines are all pretty straight. OK. And if he tilts his head up a little bit, that line is straight, too, the line between trigeminal and the uppermost cervical nerve input.
So it's an embryological phenomenon that you end up with this kind of map. All right. But now here's one little caveat to this whole story. Each dermatome actually overlaps with two adjoining dermatomes, as you see here. That's usually not depicted in the map.
Why is the dermatomal map so important in clinical neurology? Can you think of a reason? When you get a neurological exam, they test for sensation in various parts of the body. They're not just playing around.
If you get pain that corresponds to a dermatome or a few dermatomes, it could mean pressure on the spinal nerve. If someone has a slipped disc, he's going to have a hypersensitive nerve. And if it's affecting only the spinal root, the dorsal root on one side, it will go right to the midline and then go around the body to the midline in the back.
All right. So the neurologist needs to know these things and will always look for pain for example that follows the dermatome map. All right. How are they mapped? Well, I mentioned one region, the irritation of the single spinal root will cause pain in the corresponding dermatome or herniated disk.
But you also-- there's data in the literature from pathological cases where many nerves have been severed but there's a spared nerve. OK. So that's the method of remaining sensibility where the many roots have been severed but one has spared. Of course, the dermatome sensation and the dermatome will be spared, too.
The term myotome is sometimes here, a section of muscle that simply is the same definition as the dermatome. But now, it corresponds to the ventral root. It's the muscles innervated by one ventral root or one pair of ventral roots.
So they usually are pretty closely aligned with the dermatomes. And so there you see this spinal nerve in a dissection of a young human. We define local-- when we talk about a local reflex in the periphery, in terms of dermatomes. But in the CNS, we talk about the segment. Segments were originally defined just by the vertebrae.
The nerve tend to come out just below the spinal vertebrae except for the upper cervical ones. Which come on just above the vertebrae.
Now let's talk about the longer pathways. What's the oldest ascending somatosensory pathways that reaches the brain. It's actually a couple of them that are very ancient. When I was studying their anatomy, I learned that the paleo lemniscus was a cross pathway coming through spinal cord called the spinothalamic tract.
But in studying it further, I realized that there's actually an older one. In fact, that spinothalamic tract across pathways doesn't even exist in the most ancient of the chordates. And certainly not in amphioxus, the lancelets. OK.
These are the two-- if I just talk about sensory lemniscii that mediate touch sensation. We've got the two types of lemniscal pathways. These are suprasegmental pathways above the segments of the spinal cord.
So you'll sometimes hear talk about a suprasegmental reflex. A response is just like a reflex but it involves the brain in some ways, about the segments of the chord.
The oldest connections terminate in reticular formation. The core neurons of the brain stem, hindbrain, and midbrain. OK. But even both of these old pathways have some axons that do reach the thalamus.
And of these two pathways, one of them decussates and one doesn't. So let's talk about the oldest one-- spinal reticular. It's bilateral but most of the axons never cross the midline. OK. Most of them stay ipsilaterally.
They go to the core of the brain stem, almost all them terminating in the reticular formation. It's the least studied of the pathways but you always find in any study of the ascending spinal pathways, in any chordate.
You look at an amphioxus, you see most of the axon stay on the same side, a few of them reach the other side. So we know in animals what was most important for them, control of their blood pressure and heart rate, breathing rate and volume, autonomic and defensive behavioral response to pain inputs, temperature regulation, control of sexual behavior.
These had to be functions of that pathway. I can say that even in the absence of very specific studies of the functions of the pathways. Because I know that the sensory input from the body surface control these things and the animals had to have those functions even before they had a-- even the ones that don't have any other descending pathways.
So here in our simplified diagram, these axons would be the ones that belong to that pathway. And notice I do show a long here going to the thalamus. Others are terminating in the midbrain and hindbrain.
This picture was revised a little bit from the one in the book. OK. It actually could represent spinal reticular or the spinothalamic. And if we talk now about what are these most ancient animals-- I keep mentioning them. These are the main ones. Amphioxus, a group of species called the lancelets that we talked about earlier.
And then there's a hagfish and there's a sea lamprey. These are eel-like animals that are not bony vertebrates. They don't have a bony skull. It's just cartilage. And these are pictures of their brain you can see.
Hindbrain is the biggest here. There's the midbrain, here's the forebrain with the telencephalon, about the same size of the diencephalon, about the same size as the olfactory bulbs. And it's similar for the sea lamprey, which is more studied then the hagfish. A little less specialized so there's been more focus of studies on that animal.
And all of these animals all have a spinal reticular pathway. But not much of the spinothalamic pathways, if any at all. What's interesting about those three-- so I marked them here on the cladogram, is that you can trace animals in those three groups in the fossil record back to the earliest, earliest chordates.
Only amphioxus is an invertebrate chordate. The hagfishes and lampreys are vertebrates but they're not bony vertebrates. Or jawed vertebrates. The animal is bony, jaw and skull.
OK. Start here. And they include all the other vertebrates. So this is, as I already mentioned, that amphioxus has something like this spinal reticular pathway.
You can find this spinal reticular type pathway in hagfish and sea lampreys but they don't apparently have any cross pathways. They have a few axons that do cross to the other side but it's not a specific lemniscus. It's not a major pathway.
You get, though, that cross pathway in most of the vertebrates that evolve later, including both mammals and non-mammals and certainly the cartilage in these fishes.
So that cross pathway we called spinothalamic. Remember when it was discovered, people didn't know anything specifically about the spinal reticular. And it's been more studied and it's more important in humans. I'm not totally convinced of that because they just don't study people without both of these pathways.
So there they are. And this, if we look at it on this kind of diagram, this is what spinothalamic tract is like. Spinal reticular would be axons that go on this, mostly on the same side, with a few crossing over at all levels.
Here's the spinothalamic. It's a cross pathway but the decussation is at all levels of the chord. Because they're decussated immediately. At every level of the cord, you can find secondary sensory neurons with axons that crossover and ascend in the lateral columns on the opposite side towards the brain.
And a few of the axons do reach the thalamus, so you get-- it does provide input to the [INAUDIBLE].
So I want you to keep in mind we're not talking here about earliest chordates. It's a very long period of evolution in order to get there. I think it probably evolved as a specialization of the widely branch in the spinal reticular axons.
So there must have been some big advantage to crossing over, which I mentioned earlier and we'll talk about that when we discuss the hindbrain in more detail. So now I've mentioned a few times that spinal reticular, there's not a lot of research. So these are simply questions related to that research questions that haven't been fully answer.
OK. Here's another sensory lemniscal pathway, carrying information to the cerebellum. Why did the cerebellum evolve? What's the suggestion, the hypothesis that's actually fairly popular. In my reading of that literature, though, many people that study it focus on more very specific functions.
What is the hypothesis I talk about? It's very simple. We know all output, all movement depends on the motor neurons, right? So if I flex my arm, OK, every time I flex my arm, it depends on flex or motor neurons in the spinal cord.
What's causing me to flex my arm? Well, it can be caused by a result of visual input, auditory input, input from speech, for example. It could be doing a lot of different kinds of inputs. It could be input generated from within the brain.
How do we make sure that the various inputs that are coming in simultaneously, in very different parts of the body, are all causing that movement at the same time? Because it may be part of a sequence of movements. It's got to be coordinated. And that is a big problem, especially in large animals.
As they're growing, the length of these pathways is changing. And we know that axonal conduction is not instantaneous. It's not like life. It takes some time. So I believe the cerebellum evolved in order to adjust the relative timing of these pathways. There are our major inputs in these various senses.
They reach cerebellum. There's an amazing timing mechanism in the cerebellum for adjusting timing and major outputs to the spinal cord. So that's what I described in this slide and this is the pathway we're talking about. You can see it goes directly to the cortex of the cerebellum.
The basic cell types and the circuitry are highly conserved in evolution. And you look at widely different animals and you keep seeing the cerebellar structure. The layering does become more regular. You get new inhibitory cell types in tetrapods.
And also the deep nuclei that provide the output in the tetrapods aren't present before tetrapods. You also see tremendous variation and relative size, as we'll see in when we talk about some of the specializations.
Now it's generally true that the larger the cerebral hemispheres, the biggest part of our brain, 80% percent, the cerebral cortex and related structures in the endbrain. The larger that is, the bigger the cerebellum. But there are some interesting exceptions.
There are a few species of animals without the large cerebral hemispheres, in which a greatly enlarged cerebellum is found. What animals am I talking about?
AUDIENCE: Isn't it a type of fish?
PROFESSOR: Yeah. It's a fish, great. With what? It's got a specialization in the type of sensory input. It's an input we have no direct experience because we don't have it. In fact, they have a lot of cranial nerves.
AUDIENCE: [INAUDIBLE]?
PROFESSOR: Sorry?
AUDIENCE: [INAUDIBLE]?
PROFESSOR: It's not taste. That's another area where there's been great specialization, but taste doesn't lead to enlargement of the cerebellum. This sense does. It's electroreception and we spend a lot of interesting studies of electroreception.
Here I've drawn, from a published picture, I've drawn so I could simplify it a little bit and point out the main points. The enlarged cerebellum of a mormyrid fish. The mormyrids and the gymnotus have electroreceptions.
They have a number of cranial nerves that are the electrosensory cranial nerves. And the input comes in to the lateral line lobe, electrosensory lateral line lobe, which also becomes quite large. That's not cerebellum. It's a hindbrain structure. OK.
But everything shown in green there almost covers the entire brain. This is one part of the cerebellum in these animals. So we think that relative timing is used in the analysis of electrosensory input. But there is a lot of work going on right now.
It has been going on for awhile for the mormyrid fish, especially in Canada in the laboratory of Leonard Mailer, who is a graduate of this department.
And I hope he gets-- he's trying to work it out. And of course, computational work is being done also. So this is just a little about that and I point out that coordinated timing is important, even in our imagery, even in our dreams. And the entire neocortex involved in that projects to the cerebellum by way of the pons.
All right. That is all for today. We'll continue with this story. We'll be talking about-- we'll take a little break and talk about specializations and then go on to the hindbrain.