Flash and JavaScript are required for this feature.
Download the track from iTunes U or the Internet Archive.
Description: This lecture is the second of three on the neocortex and covers the functions, cell types, and connections of the neocortex.
Instructor: Gerard E. Schneider
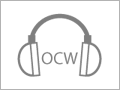
Lec 36: Neocortex, part 2
The following content is provided under a Creative Commons license. Your support will help MIT OpenCourseWare continue to offer high quality educational resources for free. To make a donation or view additional materials from hundreds of MIT courses, visit MIT OpenCourseWare at ocw.mit.edu
PROFESSOR: This is where we ended last time. We had just talked about these major types of functions of neocortex. And I've named them according to how I think it involved, location sense, object sense, functions of the prefrontal elaborations of motor cortex for planning. And I'm going to go through that a little more. And that's basically what these questions are about.
We had talked about the hippocampal system. I want you to think about how that facilitates the ability to anticipate the stimuli that you're about to encounter-- any animal is going to encounter. How does that location information that is handled by the hippocampus remember how it evolved to know where the animal is in the local environment, and eventually in many different local environments, and we remember them. Our parietal cortex remembers them, so the hippocampus doesn't have to totally relearn it every time when you go to a different area.
But because, every time you turn your head, the way that system works, this information is sent from the hippocampus back to the mammillary bodies. That means the hippocampus is getting that information based on the direction we're looking. So it can call up all the relevant memories, the things in front of us.
So it's a major factor in how the motivations make us decide to go in the one direction or another in an animal-- I shouldn't say humans, primarily. But it's for navigating the world. And that we know that motivation is a major factor. And people haven't considered enough the role of the hippocampus in motivation, and why this whole system for memory, long-term memory, evolved within the hippocampus within the limbic system, basically.
But anyway you need to contrast that with the kind of anticipatory activity that is parietal in temporal association areas or concernments. So we'll be talking more about that. So it's all in the book, and it's just a different way of looking at it in these slides where I summarize these major points.
The main, I think, anticipation, ability to anticipate stimuli, ability to plan movements, and other kind of anticipation, were major innovations of the cortex. It's not what the tectum does. It's not what subcortical systems do. This temporal aspect is so important for your cortex and, of course, planning. You know how important prefrontal areas are.
You could say that in that original multimodal cortex, the motor cortex evolved very early. Initially, [INAUDIBLE] just another sensory area. But once it became specialized for control of movement, then the areas near it became involved in anticipating movement, mainly planning it. And this is mostly theory, but I mention here support for it.
The laboratory studies of Sokolov in Moscow back in the '60s, he made extensive studies of reactions of humans to a novelty. And he studied animals as well. And how any kind of unexpected input, even if it was an unexpected lack of input, could cause an arousal response that alter the electrical activity of the neocortex. And when your inputs become familiar, then you don't get those arousal effects.
So in theoretical terms, he said that there was a central model. This is how the brain is simulating what's going on. When you're dreaming, all you're doing is using that system. Your brain is totally capable of simulating the environment in incredible detail.
Have you ever had hypnagogic imagery or vivid dreaming where you actually don't know for sure that you're dreaming? You know, you see cartoons of people pinching themselves to see if they're asleep. Have you ever had such vivid dreams that you couldn't tell?
AUDIENCE: Yeah.
PROFESSOR: Has everybody had them? Some people don't, or at least, they don't remember. But I've had them and really not very many and only when I was younger. Most of the time, I don't have difficulty knowing that I'm dreaming.
AUDIENCE: What about, like, your motivations, right? Like if you're dreaming that you [INAUDIBLE] and then you wake up?
PROFESSOR: Yeah, that's an anxiety dream. But in hypnagogic imagery, it's a good question. I don't know, we should look-- there is a literature on it, and you can read about it. And do all of the senses come to play?
You know, I remember when I had them auditory, visual, somatosensory, they were all there. I cannot remember any olfactory imagery. I'm not saying it's not possible in some people, but often it's true that some of the modalities are missing. And what you asked about emotion, that's a major issue in that kind of imagery because if it involves these association areas, the posterior cortex will generate our images of the world, the posterior parietal primarily.
The connections to emotion could be missing, and it could still generate these images. So if I have another one, I'll do the investigation. Anyway, so that same model can control endogenous generation of actions. This is the way the frontal areas and the posterior areas that do this kind of simulation of the world are connected. And you can use that model to plan your actions, and we do it all the time.
It's a view that became unpopular for a while although, back in the '60s, Don MacKay developed it. But more recently, people have come back to it and they've updated it. There was a whole issue of, I think, the journal Cerebral Cortex, one of these major journals that talks about cortex had a whole issue on this. I think I mention it in the book if I'm remembering right.
OK, so now we've not mentioned striatum in this. Actually, both the striatum and the cortex expanded in evolution including recent evolution. They can operate relatively independently, and that's because they don't operate independently in humans at all. But in animals, it's quite possible for them to operate independently. You can have an animal where much of the cortex is rendered nonfunctional, and he can still use his striatal system.
Why is that? How is the striatum then getting its inputs? Where did they get its inputs if they don't come from the cortex?
Remember they come from the polar parts of the thalamus. The nuclei we call the intralaminar and midline nuclei, they're mostly getting multimodal input. But some of those nuclei are more dominated by the visual areas of the tectum. They're getting a lot of input from the optic tectum.
The somatosensory system pathways don't all come through the tectum, some of them come directly from the spinal [INAUDIBLE]. And auditory inputs also reach those. And in addition to the intralaminar and midline nuclei, there are what appear to be older parts of the thalamus in the posterior thalamus. There's a group of nuclei sort of in the interstices between the visual, somatosensory, and auditory-- the main nuclei we consider to be involved in those three senses. But outside those nuclei there's the posterior group of nuclei, and it's basically multimodal cells that project to multimodal cortical areas.
OK, well we'll be talking more about that in a minute. But how did the cortex expand? We've talked about this before. It expanded by individual areas getting a lot bigger. Like the striate area, especially the foveal area, got quite large, like here in the owl monkey.
But also there's been a multiplication of different representations of the same sensory surface. So in this case, the retina. And it's represented in different ways. And sometimes we don't understand the functional differences, and in some cases we do understand. And that's an area that the functional imaging work in recent years has contributed quite a bit to for understanding this in the human brain.
So what was probably the earliest parcellation of the pallium? Parcellation meaning it gets divided into different parts. How did the pallium get started? The whole endbrain, initially, grew out of the olfactory system. It was totally dominated by olfaction.
This view is actually a common one. It was argued by C. Judson Herrick, for example, in the early part of the 20th century. It was commonly believed that the evidence for it wasn't major. They didn't know about some of the most primitive vertebrates. So he used the tiger salamander more than any other animal and based a lot of his conclusions on what he found in the tiger salamander.
At that time, they didn't have the really good experimental tracing methods for looking at connections. But I have the big advantage now in writing about this stuff in that the techniques are much more sensitive, and they have been applied in a number of studies to some of these really primitive vertebrates. And that's why, when we talk about olfactory system, we talked about the sea lampreys and the hagfish. You know, hagfish are more specialized. So some people don't think they're a very good model.
But in both of those animals, olfactory projections go to everywhere in the endbrain, everywhere. In fact, they even go into the diencephalon directly from the olfactory bulbs. So the earliest parcellation is separation of the olfactory inputs from the non-olfactory. That's the earliest parcellation.
So if you look at the gene expression data in the sea lamprey, it's been a little controversial with some varying results. But people that have reviewed it-- that have gone over all of it and reviewed it carefully-- will tell you that there's at least two main types according to gene expression. And it corresponds to the non-olfactory areas.
AUDIENCE: Corresponds to what?
PROFESSOR: Non-olfactory and olfactory. OK, so that was the earliest parcellation in the pallium, and we know that that olfactory cortex doesn't get direct olfactory input from the olfactory bulbs, right? Because olfactory bulbs project directly to it. But the part that the olfactory bulb doesn't project to, it's dominated by the thalamus
So then I ask about factors that influence thalamic parcellation, so we'll look at that. And then I mention what you commonly hear-- and you'll probably hear it in medical schools, if you take [? neuromatter-- ?] but these multimodal association areas are the most recent to evolve. I would change that a little bit and say, no. They were probably the oldest type of cortex, but they're the most recent to really expand in the human brain because there's evidence that the unimodal areas are evolved out of the multimodal areas.
If you look at the brains of some rodents that are a little more primitive in their structure, like the prairie voles, have been well-studied with this, and look at the cortical unit recording work. You'll find that there's a lot of multimodal input into areas that we used to think were unimodal. They are multimodal. And even in the primary sensory areas, there's often more than one modality. You find other modalities going right into auditory cortex and visual cortex, for example. All of it supporting this view. Yeah?
AUDIENCE: In the centipede, do they find like incomplete parcellations?
PROFESSOR: That is so loud in the back, I'm having trouble.
AUDIENCE: Centipedes, do they find incomplete parcellations?
PROFESSOR: There's a lot of incomplete parcellation. In fact, with some of those families, you never find complete parcellation into the sensory areas. And that is just never taught. I don't know why it's not taught.
Even Hubel and Wiesel, here at Harvard, doing that beautiful work on the visual cortex. They started with cats. They found some auditory inputs into the visual cortex of the cat, and you have to look very hard for it in their early papers. And I asked them about it one time, and Torsten told me, yes, we did find that. And we didn't know what to do with it, so we sort of forgot about it.
They were just working out how the cascade specification of unit properties in the visual system because that wasn't known yet. So they focused on that and so the auditory input just wasn't important enough to understand the main things that the visual cortex was doing. And I'm saying, well, if we're interested in evolution, though, we have to pay attention to these kinds of things.
AUDIENCE: [INAUDIBLE] was asking what if-- like if there were people who have synesthesia.
PROFESSOR: Oh, the synesthesia. OK.
AUDIENCE: Is the lack of a conversion from a multiunit area into a unimodal area part of what causes the phenomena?
PROFESSOR: Yeah, there is no evidence for that that I know of. But now with good functional imaging methods, it would be possible to study synesthesia in a way that we couldn't before. You know, it's a good question. I will look into that because it's such an interesting question, you know. But I really doubt its result in any major differences in the way the human brain is connected.
So this is just what we're talking about. And the evidence I talk about, this is actually from data from hamsters that I studied. You're looking at projections from inferior colliculus, superficial and deep, superior colliculus, and from the pretectal area. And looking at where they go and the pattern they form in the thalamus. And if you look carefully at this you see that, in the thalamus, you have the same spatial arrangement that you have this here.
The auditory input comes in in this more inferior lateral position, and that's where it forms its connections. But it's right next to multimodal parts of the lateral posterior nucleus. And then in the more superficial parts of the lateral posterior getting inputs directly from the superior colliculus, it's visual. And up in front you have the pretectal area, and those axons course rostrally near the midline. And they go right into the rostral parts of the lateral nucleus.
Highly neglected that pathway, and it's only recently that you can find studies of what it might be doing. Because I've worked in this area, I've paid a lot of attention to that. I think when this picture was reproduced in the book, they put just L. But it's usually called the lateral dorsal nucleus. A few older [? papers ?] will call it lateralis inferior.
OK, and then my interpretation of parcellation is that this shows input from the retina, here in the dark blue, input from pretectum and inferior colliculus and superior colliculus coming into the thalamus, and how, initially, it's almost certain this big tectum sending projections into what, initially, was a pretty small diencephalon. They overlap a lot.
And if you look at the brain like at the tiger salamander, you find these kind of widely-branched axons in primitive animals. In development, you still see a lot of that. But then, as they form connections, as the connections increase in density, they tended to segregate from the other axons. And that's what I'm showing through these later stages.
So here, they're almost completely segregated. Inferior colliculus going down here, the retina going more superficially in the thalamus there through the ventral part. Superior colliculus carrying visual information that did overlap in the geniculate body, but then it took over the parts of the LP. And in the pretectal, focusing on just specific sections of the LP. So you end up with this pattern. But that process where you get the segregation is called parcellation.
Experimentally, in development, if we got the retina to send projections to the wrong side of the tectum, here's what they do developmentally. They grow in, and they just overlap with each other. They just intertwine. But then as they begin to form connections, the connections of one eye and the connections of the other eye segregate.
As they get denser they tend to terminate in the areas closest to where they came in because that's where they formed denser connections first, you see. They got there first. That's where they form the connections first. And then they segregate. They pull away from each other, literally. They don't necessarily degenerate, but it withdraws.
All right. So the idea here is that multimodal convergence was the primitive state of all of the thalamus. And then parcellation occurred. And correlated changes occurred within the neocortex.
[PHONE RINGING]
I'm getting reports on a member of the family that's in the hospital.
[PHONE RINGING]
I can wait a few minutes to look at that there.
All right, so when you look across widely different animals, like, here's the [INAUDIBLE]. This is animals like the salamander or the frog. They have fewer thalamic areas. When you go up to reptiles, there's more. Birds, even more. And mammals, the most.
If you look in the human compared with other primates, you'll find the same areas, but you can subdivide the areas more. It's like the brain, with evolution, becomes more specialized. There's more parcellation than there is earlier. And that's with this comparative data support. This is just a concept based on one that [? Streeter ?] published after reviewing a lot of this work.
And then I talk about these three systems separately. These are all things that we did talk about when we talked about these systems. It's just a reminder. And then I talked about that segregation within the somatosensory system that led to the revolution of the motor cortex.
And this is the picture that I had. This was from chapter 15. And it shows how you can define somatosensory cortex. You can define it in terms of thalamic projections. The part that gets input from the posterior nucleus because that's where the somatosensory input comes in from the spinal cord in the trigeminal system.
And then, anterior through that, the ventrolateral nucleus projects, what we call, the primary motor cortex. The VA anterior just goes even more anterior, the premotor cortex.
And it shows how these projections of those two nuclei are completely overlapping in the [INAUDIBLE]. So if you record from it, physiologically, you can't separate them. If you study the projections, you can't separate them.
And then this is not the opossum, it's the brushtail possum. It's a different animal. And you find an area that doesn't overlap for each of these nuclei but then there's a fairly large area where they overlap. If you look at a rat, there's just an area of the hindlimb representation where there has not been a complete parcellation. But otherwise it's pretty segregated.
If you look at a primate, just a galago, complete segregation. That's true of all the larger primates. In fact, I think all of the primates that I know about are like that. So the evidence is pretty good for this kind of parcellation in the somatosensory system, and how the motor cortex evolved out of it.
OK, whereas the visual system, people have looked at widely different animals including marsupials. You always see a V1 and a V2. And in most of them, you see a third area that's always there, an area we call MT in the monkeys.
And then you'll see varying numbers of additional visual areas. In some there are not many at all. There's not much cortex. But in the large primates and in humans, there is a very large number.
So these are the methods of expansion that we've talked about. These are pictures of that. Primates, and here you see in the gray color here-- maybe that's a blueish gray-- you see what [? Nessalum ?] calls the heteromodal areas, the multimodal areas.
And it's typical to picture rat and hedgehog, especially hedgehog-- you see how they picture it-- no multimodal areas. It'll be interesting to see how my colleagues that have published these kinds of pictures for a long time will react because I've looked really carefully at that literature, and that is not what you find. There's a lot of multimodal cortex, and this is-- so I have this line, multimodal regions do exist in between the major sensory areas in hedgehog, hamster, vole. It's been best studied in the vole. And unimodal and multimodal association areas are not so distinguishable as they are in the primates because they're all multimodal, and these two modalities will go into those areas. They might be dominated by one sense, but they're not really unimodal association areas.
And then I talk about some multimodal inputs even in the primary sensory areas. And that just indicates that this parcellation isn't complete. Maybe in the large primates and in humans, it's about as complete as it can be. But not in many animals. So it's a neglected fact that gives us clues about evolution. And because of that I've paid a lot of attention to it.
And then I have a little bit about axon trajectories that you should realize. This is for reptilian cortex, which you'll see the same thing for amphibians and fishes that where, in the cortex, the axons don't come in from below. Like in this picture, you'll see here for the hedgehog, the mammal, they come in through the white matter. Then they go up and terminate in the various layers including layer one there.
Whereas in the reptiles and turtles and in the salamander and other amphibians, they come in like this. They don't come in through a white matter, they travel conventionally. So if you look, this is the way that it was pictured. And Allman, he used a picture here of Pedro Ramon y Cajal, Santiago Ramon y Cajal's brother.
And this is from the American, CJ Herrick, where he shows a similar level where you see this beautifully thick medial pallium with this cortex next to it. It's a multimodal area of dorsal cortex. In most animals it's called dorsal pallium or dorsal cortex. And it projects heavily into the medial pallium, but it gets multimodal input from the thalamus.
But you see how the axons travel. Here they come out of the lateral forebrain [INAUDIBLE] they travel. They don't travel through a white matter at the base like the mammals.
OK, so then I point out that in the hamster, and this is true for hedgehog too, there are some neurons in the lateral thalamus. Some of the data indicates they might all be in the posterior nuclei group, but they're in the lateral thalamus. And they have a trajectory that's more alike those primitive animals. So here's, I call it, the type one axon coming in like from geniculate body and arborizing primarily in layer one and four. But they have some branches in the other layers as well.
But then you have this other type that travel long distances and terminate-- it's as if they ignore the boundaries between cortical areas. They go-- even the primary visual cortex apparently carry multimodal input. It's an odd fact in neuroanatomy that such axons exist, and they have not been studied very well at all. OK, but they can account for some of the physiological results that we've been talking about.
So I want to talk more specifically now about-- this is now stuff from chapter 33, which we'll talk about next time as well. We'll try to finish chapter 33 next time and maybe at least get started in the last chapter in the book.
So I wanna say more about cell types and they're connections. Different regions-- how we name them-- there's different ways to talk about different regions. And then the major fiber routes which we brought up before, but I want to review them. So you should be able to contrast some of the major neural cell types.
What are the two main cell types that you can think of if you just think of structure and talking about neocortex? When [INAUDIBLE] talked about these, he just mainly talked about these two cell types. The dominant cell type, parameter cells. With the apical dendrite going right up towards the surface and arborizing, OK. And the other type, you can lump them all together and call them stellate cells.
There are other shapes-- a fusiform shape, for example, sort of a bipolar-looking neuron. But there's other ways to classify them. You can use neurotransmitters. You can use whether they're spiny or non-spiny, whether they're excitatory or inhibitory.
So the criteria vary. And the nicest classification I've seen is in this picture, which I've put in the book, in which they separate these groups according to the spiny excitatory neurons all using glutamate as a transmitter, the non-spiny inhibitory or GABAergic interneurons. And notice that these are most all stellate in shape, a few of them might have a fusiform shape, but mostly the star-shaped cells that don't have an apical dendrite.
Here are the pyramidal cells, with this apical dendrite. And this you see here, this is one in layer five. It's a big pyramidal cell. The apical dendrite goes right up and arborizes in the layer one.
And these pictures lack a lot of the processes that are really there that are pretty complex. And it shows an enlargement here. You look at higher magnification of a Golgi stain or a cell that's been filled with the HRP or something like that, you'll see the little spines they can take. Sites that many of the synapses are formed in are spines.
And then it shows where they go. Like these big cells in layer five go to the spinal cord, brainstem thalamus, striatum, and others of cortex, OK. The pyramidal cells in layer three and layer two are much smaller, but they're still pyramidally shaped. They're transcortical, so they're association cells of the cortex. The [? colloquial ?] cortical cells. A terms that's not usually used, but it's an appropriate term.
And the only excitatory cell here that's not pyramidal in shape is a little stellate cell. We happen to call them granular cells in layer four. A lot of input from the thalamus comes in here. And the axons simply terminates within the same column, carries that information right up to the superficial layers, which we often call association layers, layers two and three, because they contain these neurons. They're getting input from these stellates and therefore they're projecting others areas of cortex.
Now they do get other inputs. They get some direct input from the thalamus too, because the thalamus contacts those branches of the pyramidal [INAUDIBLE].
And then you'll see here for the non-spiny inhibitory interneurons, how many different shapes. And look at the way the axons form. You see cells like this that have an axon that distributes all the nearby columns.
Here's one that all the axon distributes up and down the same column. They've all got local axons. Some of them covering a number of columns. Some of them covering only one column.
But so two major divisions according to this classification, but if you do it just structurally you would say pyramidal and stellate are the two major types. And here's from [INAUDIBLE] book. He shows a small pyramidal cell in layer six, and then he shows a couple of stellates. And he has a more elaborate picture of both the dendrites and the axons.
Whereas other anatomy books, like Brodal has a simplified picture of, in this case, interneurons where he's showing some of those same cells that are in the other picture. But he's put the axons in red like they did, simplified the dendritic tree a little bit and indicates how they terminate. These are all GABAergic cells, except this one. This is probably an excitatory cell therefore. So this would be the only one that is classified over here because it's a spiny excitatory cell.
All right, and then I point out here that not all local interconnections within the neocortex are made by the short axon interneurons. And I'm talking about just locally. In the same column or between one column and nearby columns. They're made by axon collaterals.
Almost all pictures that you see in textbooks never show this elaborate collateralization of the axon. But the Scheibels were very good anatomists. They published some of the best Golgi pictures in modern neuroanatomical literature.
And here they're showing that the dendritic spread in the cat of neocortical cells averaged around 500 microns. Whereas the axon collaterals, collaterals of the initial segment of the axon, in this case, the axons are all going out. This one to other cortical areas. These two to the thalamus and brainstem and spinal cord. Look how much they cover, three thousand microns so three millimeters. So huge numbers of local connections are formed by these axon collaterals.
Now you should be able to answer this, how neuroscientists define cortical layers and cortical columns. Divide it into anatomical methods where then this whole method and also the Golgi method, if it's so dominated, also [INAUDIBLE] stains. But what about the functional side, electrophysiology, our initial definition of columns came from electrophysiologists putting microelectrodes and seeing functional differences between one regional cortex in the immediately adjacent. Like in the visual cortex, you'd find that cells here respond to one eye, cells here respond to the next eye. And then back to the first eye and the second and so forth.
So physiological methods and various anatomical methods. There are molecular methods in addition that have been applied, especially for layers. You look back in chapter 2, I had a picture where they had antibodies that bound to cells in specific laminae, specific layers of the neocortex. So that's still one more method.
Molecular methods used for neuroanatomy. And this just summarizes these pictures. This is the one I used in the book, and this is the one that usually you see in books.
And people will wonder, why did I choose this one by an American neuroanatomist and not the one that's always published? And that's because I insisted, with Amy's help, finding the sources. If I found people cited a certain source, I found the source. And I found out it was wrong again and again and again because people usually aren't that careful in their scholarship.
I just wanted to point out to you that you can't believe everything you read even in science. Especially, scientists aren't always very good historians. Just thought you might like to know that.
And we'll have to stop there. We'll come back and go through a few of these things. Now focus on the pictures. Make sure you're understanding the pictures, OK. They're all posted.
It talks about cortical types, cortical columns, how you can define them anatomically, layers as well, and a few of the things that are commonly stated. OK, so we'll come back there in the next class. And I know we can get through these, and hopefully, we can get a little bit into the next chapter as well.