Flash and JavaScript are required for this feature.
Download the video from iTunes U or the Internet Archive.
Description: This lecture covers the layout of the visual system and the retina as well as photoreceptors.
Instructor: Peter H Schiller
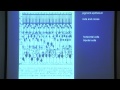
Lec 2: Basic layout of the ...
The following content is provided under a Creative Commons license. Your support will help MIT OpenCourseWare continue to offer high-quality educational resources for free. To make a donation or to view additional materials from hundreds of MIT courses, visit MIT OpenCourseWare at ocw.mit.edu.
The first thing I would like to tell you, which I did not mention the last time-- that in the reading assignment, the syllabus that we handed out-- and by the way, does everybody have one? If you don't, we have some extras here. You all have one? Good.
Anyway, as you can see on the first page, there is a section called preparatory reading. So I would recommend that if you can each time read this recommended reading, which is available, of course, on the internet. And that will make it easier for you to follow the lecture, because this course is both in vision and audition, and relies on imparting a great many facts about the workings of these two systems. And it's easier to be able to memorize these facts by doing the preparatory reading, if you can.
All right, so today, then, what we are going to do is initially start to talk about the layout of the visual system. And then we're going to talk about the retina for much of the course. At the very end, we'll talk a little bit about the lateral geniculate nucleus.
In each case, I will try to tell you about how some people thought about things, and what the progression is of the discoveries that have been made about the visual system. It is certainly an area of research which has been incredibly successful, and has resulted in quite a number of Nobel Prizes for the discoveries that individuals had made in uncovering the workings of the system. Furthermore, many of the uncoverings that investigators had done came about as incredible surprises, because so many of the findings were quite unexpected. And I will try to point these things out during the course.
And my section, as I mentioned before, will consist of 12 lectures. And then that will be followed on October 23rd by a midterm exam. And again, to reiterate, that's going to consist of a series of multiple-choice questions. And then following that, we'll move on to talk about the auditory system that Chris Brown is going to be presenting to you.
All right, so the first thing I want to do, then, is to talk about the basic wiring of the visual system, which in itself has yielded a number of unexpected surprises, and also raised some interesting questions. So what I want to start with first of all is that when you look at some animals that have sideways-looking eyes, like the rabbit and most amphibians, the two eyes look out in two different directions-- to the left and to the right. And that enables them to see a very large portion of the visual field.
And when that is the case, as in this picture shown here for the rabbit, what you find is that there's only a small area which is seen by both eyes. But then when we look at higher mammals, and particularly primates and humans, what you find is that there was a huge change in the course of evolution, bringing the eyes to the front. Now that change is very interesting, and we can ask, why on earth did that happen?
Because it meant that, obviously, a human cannot see behind themselves, where these rabbits can see a much larger portion of the visual field. And so you sacrificed ability to see all the way around the world for being able to see with both eyes to the front. And so the question is, why did this happen?
Now we are going to discuss this in some detail, because one of the prime reasons-- I believe at least-- that this has happened is to be able to process information about depth better than it can be done by rabbits and other animals. And so when we talk about depth perception, we are going to deal with this issue in more detail. Now if you look at an animal like a rabbit, what you find-- and also animals which are, as I've mentioned already, that you also have in fish and amphibians, you have these sideways looking eyes. And now we can ask the question, how do these two eyes make their connections through the ganglion cells to the central nervous system?
And that, in fact, was quite an issue at one time, believe it or not, before anatomical techniques became more sophisticated. And the reason it became an issue is because it was discovered predominantly, actually, by Cajal, who I've mentioned had received the Nobel Prize for the beautiful work he had done on not only vision, but in general about the nervous system, in 1906 with Golgi, he discovered, as did several other investigators, that the optic nerve crosses over at the so-called chiasm. And so the input from the left eye projects into the right half of the brain, and from the right half to the left half of the brain. And that became a huge issue-- why on earth would this happen?
And we are going to deal with this repeatedly. It's a very interesting issue. And even more interesting is the fact that when the eyes move to the front, what happened is that this kind of connection became much more complicated.
But before I do that, let me just say a few more words here. First of all, that in fish and amphibians, you have a huge structure called the optic tectum, which is the primary visual processing center in the brain. And there is also the lateral geniculate nucleus of the thalamus in these animals. But that's quite small, and not very well developed.
And there is a cortex in these animals, but it is, again, a primitive area compared to primates, for example. And the lateral geniculate nucleus projects to the cortex. So the left cortex in these animals gets input from the right eye, and the obverse is the case for the right cortex. And so consequently, this cortex is this part of the visual field, and this cortex is this part of the visual field. It's almost like an inversion.
Now we come to what happened as a result of the eyes moving to the front. When the eyes move to the front in the course of evolution, that necessitated a major rewiring in the visual system. So that's what we are going to look at next, because that's us.
All right, so what we have here then, we have the two eyes looking to the front. And imagine that these two eyes are looking at something. There's a dot here. And both eyes are looking at the same dot, so that you only see a single dot. OK.
So now what happens is really something surprising. What happens is that the-- let me interrupt for a second and tell you that imagine that you cut your eye-- I mean, don't do it-- but imagine that you cut your eye vertically in half, each eye, OK? And that means that each eye has, if you will, a nasal half and a temporal half, all right?
So here we have the two eyes. Here's the left eye. And what we have is a nasal half, projects across, and the same thing is true for the right eye-- projects across. But now if you look at the temporal hemiretinae-- hemiretinae, remember that's the word for it-- something very interesting happens. The temporal hemiretinae don't cross over, but they remain on the same side. So we have a color code here that the red parts project across, and the black parts also project across from one eye, but stay ipsilateral for the other eye.
So you say, my god, why did this happen? What on earth is going on? And then if you study this, you realize that this is actually one of the few truly logical things that you encounter when you study the brain. Many of the things you study in the brain don't seem to be too logical, because in the force of evolution, you have to change things in very peculiar, subtle ways to make things work. You couldn't just redo the whole system from scratch. But this is certainly a logical one, and I'll explain it to you in just a minute.
OK, so now what we do next is that again, we have the two lateral geniculate nuclei, but now these are in the thalamus. These two nuclei have grown tremendously in size, and became much more important in visual processing, as did, then, the cortex. This process, by the way, is called encephalization. So in primitive animals, they have a very small cortex. And then the course of evolution, the cortex grew, and grew, and grew, and grew, and became a more and more important structure in being able to analyze just about anything, and certainly analyze vision.
Now here we have a cortical area in the posterior part of the brain, as I pointed out to you the last time. And what you have is a whole series of visual areas in the cortex. The most central one, I guess, would be area V1-- the so-called primary visual cortex to which the lateral geniculate projects most profusely.
Then we have a whole bunch of other visual areas, as we'll discuss in more detail later on. And then what happens is that we have several other cortical areas. The cortex-- I think you all of you know that-- is divided into four lobes, OK? The frontal, temporal, parietal, and the rear one is called the occipital lobes. All right, now the visual areas that you see in the back here make extensive interconnections and connections to these other lobes in the brain to be able to analyze the visual scene.
Now in addition, what we have still-- as we had in more primitive animals-- we have a superior colliculus-- actually two of them, one on each side-- and then we have another set of areas called the terminal nuclei, the nucleus of the optic tract, about which we will just talk a little bit, because they are specializing in certain visual functions, but not nearly as compelling and interesting as the work that has been done in the cortical areas and in the superior colliculi.
So this is the arrangement. But now we want to understand, why do you have this strange connection here? So to understand that, what we are going to talk about is the so-called horopter or the Vieth-Muller circle. Now what is that?
Some clever guy came up with this observation. They made a circle that goes through the nodal point of the eye. And its diameter depends on where you're fixating. So fixating here, in this case, the red area, which is your left visual hemifield, impinges on the nasal retina of the left eye and the temporal retina of the other eye. So if you have an object here, that hits corresponding retinal elements in the two eyes. And that is a basic rule.
Anywhere along this horopter, a spot will activate corresponding regions in the two eyes. And then what happens is that those corresponding points go to the same location in the lateral geniculate nucleus, and in the superior colliculus, and in the visual cortex. So the logic of this arrangement is to be able to have the two eyes have input to corresponding points in the brain. That way, you can see something uniform and clear, rather than seeing double.
So that is, then, the arrangement that you have in primates. Now just to anticipate a little bit-- whenever images fall inside or outside the circle, they do fall on non-correspondent points, and that will have something to do. And I keep you just curious about that for the time being, about how we process depth. And that is a mechanism that is involved, so it's called stereopsis. I've mentioned that very briefly the last time.
OK, so that then summarises the basic connections of the system. And then what we are going to do next is we're going to go to the retina, and look at in more detail about how the retina is made. And then we will go in successive steps to higher areas, sometimes going back and forth, to try to understand visual processing in general.
All right, so now let's begin by looking at the eye. Now I don't expect you to know all these names here, but you should remember, of course, the lens, the cornea. You know that already. You know the iris, right? What's the iris?
AUDIENCE: [INAUDIBLE]
PROFESSOR: OK, now what do we base our views on the color of the eye when you say somebody has blue eyes, or somebody has brown eyes? It's based on the fact of what the coloring is of the iris, all right? Now, what's the iris? The iris can get bigger and smaller to control the size of the opening into the lens. All right?
It's much like what you have in cameras, where they have the so-called f-stop, right? So if you have a large number, say f/16 on a camera, that is set to 16 if you do it manually when it's bright out there. But when it's dim out there, like we have in this camera here, we have the lens wide open because it's pretty dark in here. Then you have a low f-stop, like an f/4 or even lower, to allow more light to come in-- more photons to enter the eye.
Now next is-- here's the lens. And the fascinating thing about the lens is that in the eye, the lens is totally different from the way it is in a camera. Now, maybe by now many of you don't even know the details about a camera, but one of the details, of course, is that to bring an image into focus, you have to adjust the distance of the lens from the film onto which the image projects, OK?
So the closer an image, the further out the lens has to go. Now, if that were the case in animals and humans, you would have a super-bulging eye, so when you look at something closely, the eye would go out like that. And that would be really disturbing. So that is not the case, because what happened instead is something very clever that you don't see in cameras, which is that you have this lens here, which is-- at least in younger people-- is an organ that can vary in thickness.
So you have here some muscles, the ciliary muscles, which are just the thickness of this lens, to allow it to focus properly on the retina. Now here, the retina goes around, is against the inner wall of the eye. You all know that, of course.
And to explain how this is done, then-- very, very crudely-- here we have it. If you have an object really near, you want the lens to be thick to properly focus the image on the retina. And if the image is far, you have to thin the lens to get the proper focus on the retina. So that's how the lens works, at least when you're reasonably young.
But then when you get older, what happens is that the lens becomes progressively stiffer and stiffer. And because of that, you have to start wearing glasses. Now, in the olden days, they used to have lenses which came in two parts-- bifocals. So if you looked in the upper part, you could look at a distance. If you looked in the lower part, you could look close.
Nowadays, they have graduated lenses, so if you look in the upper part, you can look far. And if you want to look [INAUDIBLE] look down below, and then you can see that in focus. So once you get older, these lenses don't adjust as much-- still a little bit. But the proof that this is not that important, thank god, now that we have these graded lenses and we have bifocals-- is that a lot of people in old age, who develop-- and sometimes not even in old age-- in India, for example, there are a lot of young kids who have cataracts.
Now what are cataracts? Cataracts interfere with the processing of vision through the lens system, and things become unclear, because all kinds of blockage occurs in the lens itself. So how is that corrected? The way that's corrected nowadays, is that you have what is called cataract surgery.
So what they do, then, is they actually literally remove the lens from the eye, and they put in a fixed lens made out of plastic or glass. And then, obviously, since there's no adjustment, you do have to wear glasses to look at things far away and look at things close. And most of the time, those would be graduated lenses. And so people who have cataract surgery can see extremely well, certainly a lot better than having to try to deal with lenses that have cataracts.
All right. Now there's one other point I want to make about the eye here before we move on-- maybe two points. One is about this part of the eye-- that you have here your so-called iris, OK? And what do you call what's in the center of the iris?
AUDIENCE: Pupil.
PROFESSOR: Pupil, very good. Now what is the color of the pupil? In most people, it's black, right? Why is it black? How come the pupil is black? Any thoughts about that?
OK, well, let me tell you. And I think what I'll do is actually I'll move on. First I'll leave this unanswered for just a few minutes. This I've shown you before, which is another amazing thing about the eye-- namely that in the fovea, you have something like 200,000 cones per square millimeter-- 200,000. As you go five degrees out, it reduces tenfold to 20,000. And then you go 10 degrees, it reduces by half.
So because of this very high density-- I've mentioned this before-- in the fovea, you have high acuity. And that is one of the prime reasons you have to move your eye around so that you can see things in fine detail. All right, so now let's take another step here, and look at the retina itself.
And let me first of all point out to you, I have reversed the image to have it right-side up. The light actually comes in from the bottom, and goes through all these cells until it hits the photoreceptors. Here are the photoreceptors. And at the closest point to eye, what we have is called the pigment epithelium.
OK, so what's the pigment epithelium? The pigment epithelium is a single layer of cells that are pigmented to such a degree that when photons come into the eye and get to the pigment epithelium, they get absorbed just like a black surface, that you see as a black surface, absorbs the photons and doesn't reflect them. So because of that, there's no reflection back out. And that explains why the pupil looks black, because the light that comes into the eye doesn't reflect back out.
Now there's a good reason for that, because if it were to reflect back out, it would scatter, and it would activate many more photoreceptors receptors than it should, and because of that, you get blurred vision. Now how do we know this? Can you think of any one species of animal where the pigment epithelium is not black?
AUDIENCE: In cats.
PROFESSOR: OK, in cat, OK. So there's some animals, you see mostly in animals that specialize in nocturnal vision, that have a pigment epithelium which actually has purposefully reflecting molecules in it. For them, this is called, actually, the tapetum. And the purpose of that is to improve the ability to see so that the photons activate the photoreceptors not only in one direction, but also when they bounce back.
Now the tapetum is arranged in such a way, in most animals, that it doesn't freely scatter the light, but scatters in a fairly directional manner. And because of that, you can see pretty well. Now I'm sure that many of you have noticed that when you drive at night, in a the deserted area in the United States-- which are decreasing, of course-- you can see sometimes deer on the highway, or rabbits for that matter. And when you see them, it looks like they have two flashlights projecting at you. It's almost scary. But it also helps you by being able to avoid hitting them. Now that's because they have that reflecting tapetum, all right?
Let's look at a different example. What other human can you think of who does not have a black pigment epithelium or for that matter, some animals as well. Yes?
AUDIENCE: Albino.
PROFESSOR: Very good-- albinos. Now what's albinos? Albinos are individuals, or animals, who lack pigment in their skin, and, of course, in their retinae. So if you look at the eye of an albino, actually their pupil is not black. Their pupil is--
AUDIENCE: Red.
PROFESSOR: Reddish, very good. Why is it reddish? Because you have thousands of-- in the retina, especially mostly on the outside here as well, you have blood vessels. And the blood vessels, since they carry blood, when light reflects from them, they will have a reddish color to them, and thereby endow, if you will, the pupil with a reddish color. The pupil with a reddish color.
Now, the proof that the main function of the pigment epithelium is to absorb the incoming light, to prevent scattering, is in the fact that albinos that lack this pigment epithelium have very poor vision. They often have vision to 20 over 200 or something, because the light scatters from the epithelium which is no longer pigmented in these people, and activates many photoreceptors, thereby reducing the ability to see things clearly and sharply.
All right, so now let's go through this retina here. First of all, we have the rods and the cones. Now this brings me to a very interesting story-- at least for me an interesting story.
Back in the 19th century, there was a person whose name was Max Schultze. I'm trying to remember when he lived. He lived from 1825 to 1874. He developed some new anatomical procedures. And as I mentioned before, whenever new techniques emerge or are invented, it's likely that you're going to make some interesting new discoveries.
And so he was the first person, by then looking at the retina and looking at the photoreceptors, that he said there are two different kinds of photoreceptors-- the rods and the cones. The rods are sort of, like the name implies, rods. And the cones are sort of, as the name implies, conish looking. So he looked at that and looked at that, and said why on earth would we have in most animals rods and cones? What is their function?
This was a totally new discovery. When he first published this, nobody believed him. They said, oh, they just vary randomly, and some are more rod-like and some are more cone-like, but it's just the same photoreceptors. So he, of course, did not accept that. He saw, by being very careful about it, that it was definitely two different classes just like it is in this picture.
And so he began to ask the question, why on earth do we have this? So imagine yourself in the 19th century coming up with this incredible discovery, and trying to figure out why we have them. Now if you're a really good scientist, then you start to think analytically. And so what did he do? I'm so impressed to this day.
He said, well, let me take a closer look at the retina and see how the rods and the cones are distributed on the retinal surface. And he discovered, to go back to the previous slide, that there is a small region here, the fovea, where there are only cones. There are no rods. And I'll show you a better picture of that in a minute.
And he said, my god, if that's the case, there must be something different about the way we see in the fovea than we can see in areas where you have lots of rods. So what did he discover? He discovered that in the fovea we don't see too well at night. OK, on the basis of that, he concluded that the rods are for night vision.
Now this was absolutely incredible. There's only one sad part in this story, which is that this discovery was made in the early 19th century, but before the Nobel Prize was initiated, because had the Nobel Prize been initiated before you made this discovery, there's no question that he would have received the Nobel Prize for it. It's such an incredible magnitude of discovery.
All right, so let's go back here then, and I pointed out the rods and the cones and the pigment epithelium. Now let's go through here and I'll tell you about the rest of the elements. And then this time, and also in several subsequent sessions, we'll talk about some of these elements in much more detail, especially about the retinal ganglion cells.
So anyway, when you come here to the next stage here, we have the so-called horizontal cells and bipolar cells. It's been established that the photoreceptors connect with both of these. And then the bipolar cells connect into the inner portion of the retina, just as I'll show you in a minute.
Now this region here is called the OPL, and it's easy to remember-- outer plexiform layer. Now then, when you get into the deeper parts of the retina, you have the so-called amacrine cells and ganglion cells, and they reside in the inner plexiform layer. Now it is the ganglion cells that project out of the eye and then project, as we had shown you, to various regions in the brain, most notably that we're going to talk about today the lateral geniculate nucleus.
Now let me also point out at this stage to you some numbers. Here is an enlarged, highly simplified view of rods. Now, the rods are constructed in an incredibly complex way. They have these individual so-called disks. And within each of these disks-- there are about 1,000 of them-- I only have 25, here, I think, or 24. There are about 1,000 of these in each rod, OK?
And within each of these disks, you have about 10,000 rhodopsin molecules. There are several kinds of opsins, so-called. Rhodopsins are the ones you see in rods, in there are different kinds of opsins in cones. And these are the photosensitive molecules in your receptors, so that when a photon comes in and hits one of these molecules, they change shape.
And the simplest way of thinking about this is to say that these molecules, these rhodopsin molecules, come in two different shapes. And these two different shapes are what we call, very simply, those that are open and those are the sort of closed, if you're describing the molecular shape. And the easiest way to think of them as that they're bleached or unbleached.
And so there are millions of these molecules, and what is that, 10 million in each rod. And you know how many rods there are in the average eye? About 120 million, and about five or six million cones. And you're talking about unbelievable numbers. It's just hard to comprehend.
But at any rate, the molecules here, then, are two different states-- bleached and unbleached. What that means is when a molecule shifts from being unbleached to bleached as a result of a photon hitting it, that changes the overall-- I'm talking about, of course, hundreds of molecules, typically-- the overall degree of de- and hyper-polarization of the cell. And that will then determine whether the cell is going to communicate to the next elements below, and I'll describe that in much more detail in just a minute.
But it gives you an idea of how unbelievably complex this is. Yet another complexity is that you have this huge number of rods, but if you think about it, will these rods be there in each in these rods with each of these disks persist for a lifetime? So the average lifetime is 80 or 90 years. Well these same guys exist in the eye all the time?
Well, the answer is no. What happens is there is yet another process, amazingly, that replaces in steps these disks in the rods, usually a few disks every few days. So it's a constant, dynamic process keeping your rods, if not your whole body, young, so to speak. All right, so anyway, this gives you sort of a crude sense of the complexity of this system.
But now we are going to shift over, and talk about the retinal ganglion cells. And then we'll come back, and we talk in a bit more detail about the preretinal ganglionic elements. Here is a set of pictures created by a wonderful scientist called [? Poyak ?], showing you different classes of types, if you will, of retinal ganglion cells.
The top row here have been named, because of their small size and the small dendritic arbors, have been named midget cells. Whereas these ones below-- some of them, at least-- are much, much bigger and have much larger dendritic arbors, as you can see here, have been called parasol cells. And why they've been called parasol cells I'll explain to you in just a minute.
Now this set of pictures was created by the Golgi stain that I mentioned to you before, that was invented by Golgi, and extensively studied by Cajal. And for this incredible work they had done, a lot of it on the retina, they received the Nobel Prize in 1906. So what this disclosed, then-- an initial view-- was that there are different classes of retinal ganglion cells.
And so therefore, the big question came up, why do we have different classes of cells like that, retinal ganglion cells? What do they do? Well, initially some people again-- which is good-- I think one should be resistant to change and new ideas until they are really proven well. Some people argue that [INAUDIBLE] just continue, but then it became evident that these are indeed different classes. And I will present some evidence to prove that.
So there are actually quite a number of different classes of retinal ganglion cells that perform different jobs. We're going to try to understand what are the different jobs that they perform. And we're going to concentrate mostly on these midget and parasol cells, because they are by far the most numerous in the retina, and make a major, major contribution to overall vision.
All right, so now let's look at the physiology of retinal ganglion cells. Now that we know we have these different kinds of ganglion cells, how do we find out what they do? Well what was recognized in the early portions of the 20th century was that to find out what these cells do, one needs to study their activity. And to study their activity, what was developed after many different trials, is to either record from individual axons, or to use a microelectrode that you could put into the eye, and record from individual cells, and see what they do.
Well, the first person who did this was Keffer Hartline. And for the beautiful work he had done, he received the Nobel Prize in 1967. Now what he did-- he was a tremendous surgeon. And so what he did-- he did this in primitive animals like frogs-- he would take the optic nerve, and he would dissect one fiber from the optic nerve, hook it up, and record electrically from it. And then what he would do, he would shine a light into the eye, move it around until this cell generated action potentials. That was just a very small region, because as I've mentioned, each cell in the retina-- each ganglion cell-- only sees a teeny, teeny, little portion of the visual field.
So that's how he did his experiments. And he made some really remarkable discoveries which further then were elaborated by using slightly different methods. A slightly different method, first of all, was to put a microelectrode into the eye. And I've mentioned that to you before. The initial microelectrode was a very fine, tube of glass which was heated and then pulled until the tip was only about a micron in diameter, which enabled you to record from individual cells in the retina, or for that matter, anywhere in the brain.
Now another change in the methods that emerged is that instead of shining a light directly into the eye, people use reflected light, because in the world most of what we see is reflected light. Of course, nowadays this has changed a little bit, because we have computers and we have TV, which were set up in such a way as to mimic reflected light, if you will. But in the natural world for the most part, we see reflected light.
All right, so when this was done-- this initial work that was done by Keffer Hartline for which he received the Nobel Prize-- that he discovered three major classes of cells based on that recording of this, which he called, first of all, on cells, secondly off cells, and then thirdly on-off cells. Now the on cells were those which, when you shown light into their so-called receptive field area, they would discharge vigorously. You turn the light on here, and you can see the on cell fires.
An off cell, on the other hand, fired when you turned off the light using this particular method. And on-off cells were those that fired both at the onset and the termination of the light. And that's why they were called, and are still called to this day, on and off cells.
So that was an incredible discovery, and has created a major evolution in the study of the visual system. All right, so now doing this kind of work in more detail, using especially mostly reflected light, several other major discoveries were made. A fellow at Harvard in his later years, originally at Johns Hopkins, did experiments carefully studying the receptive field structure of these cells. He would record, in this case with microelectrodes, from a single cell-- retinal ganglion cell-- and then see how it responded when he fiddled around with the light in the receptive field.
So imagine, then, that the eye is fixed. It's looking out. You're putting an electrode, in this case, into the eye or wherever you put it, and then you can use lights, maybe like a projector, move it around to find out where the receptive field is. And then you can present a small spot of light, make it a big spot, make it different colors, and so on, and so on.
So when this was done, a remarkable discovery was made by Kuffler, namely that the so-called on and off cells were not just responding to the onset and termination of light. Instead, what they did was they responded vigorously to a small spot in the center of the so-called receptive field, but if you used a large spot like that, there was much less of a response. And that was true also for the off cells, and for the on-off cells. So the surround you can think of as being inhibitory with respect to the center.
And so the big question arose, why on earth did this complex arrangement evolve of having cells that have not only an excitatory center, but also have an inhibitory surround? And now I'm going to let you think about this for a minute, and eventually I will tell you as to why we have this organization. Because we're going to devote a whole session to the on and off systems, and also a complete session to the midget and parasol cells. And that's when we are going to discuss this in detail.
Now, the important thing as you learn about these things is to be an active thinker. And if something comes up, you say, well, why did this happen? Why did that happen? Why is this? Why is that? When you actively think about that, then it becomes interesting. And also, once the answers come, it becomes insightful and exciting to understand it.
OK, so now a series of investigators did experiments in which they-- this sounds like a fairly simple experiment-- in which they did labeling of just the cell bodies in the retina, and did what is called a whole mounts. What's a whole mount? You take the retina. You flatten it out. Then you look at this whole layout through a microscope.
And if you label these cells-- and this was a so-called Nissl stain that stains the cell bodies, but doesn't stain the processes like the dendrite of the axons. What they found was-- they did this quantitatively-- they could distinguish three very distinct different classes of cells. It turned out later that there were more than three, but in this case, just doing it crudely like that, you can see those big cells-- that one, that one, that one, that one, and so on-- maybe one out of seven or eight in this sample-- are these huge cells. And then you have some smaller cells. And then you also some tiny cells.
And if you did this quantitatively, they argued that they formed distinct separate populations. They were not a continuum. All right, so then that, of course, is a basic requirement to prove that indeed, these are different classes of cells that probably perform different jobs.
So what can you do to prove this further? Well, subsequent to this kind of work, people began to look at another interesting question about how these retinal ganglion cells send a signal to the central nervous system. And it's been discovered, not just in the retina, but in many other parts of the human and animal body, that the rapidity with which an axon can send its action potentials down from one location to the next-- from the cell body, say, to the axon terminals-- very much depends on the size of the cell and the size of the axon.
So if you have a small cell and a very thin axon, the conduction is slower than if you have a big cell and a big axon. So if that's the case, people argued that if you were to take the optic nerve and stimulate it at one side and a little bit down, maybe back towards the retina, record and see what kind of overall general-- not action potentials, but overall activation takes place.
And when that was done, a very amazing discovery was made. In this case, you can see we have two major dips here. This is time, OK. Here is electrical stimulation. Here's the activation of hundreds and hundreds of fibers.
And it shows that they formed distinct groups-- a very rapidly conducting group, a slower conducting group, and a very slow, more diffuse group. So then it was subsequent-- I'll talk about that later-- it was subsequently established that these very rapidly conducting axons come from the so-called parasol cells. The medium conducting ones come from the midget cells, all right?
I'll tell you exactly how that was established, but now that has become a solid fact. OK, so now we go back to the anatomy of these cell types, if you look at them at equivalent distances, over eccentricity, one, three, and 5.7 degrees, here are examples of the midget cell dendritic orbits [INAUDIBLE] looking straight down at the cell.
You can see these are very constricted dendritic orbits. Now by contrast, the parasol cells have much, much larger-- three times larger in diameter-- dendritic orbits. And now I can tell you-- which I deferred before-- is that if you look at these, the reason they're called parasol cells is what? Because it looks like an umbrella. OK.
So this looks like an umbrella, and of course that's parasol. So that's why they are called parasol cells. And these guys, much less interesting, are called midget cells because they're midget. They're small.
All right, so that then established-- this work was done just 20 years ago-- it was established that you have indeed these two very, very distinct populations of cells. So now the big question came up, why do we have on and off cells? Why do we have midget and parasol cells? And as I say, we are going to discuss this in considerable detail.
But let me just give you a crude introduction. If you look at the parasol cell-- obviously, because of the huge dendritic orbit-- they have large, relatively speaking, much larger receptive fields-- three times bigger than midget cells. The midget cells are so specific that each cell in central retina gets input for the excitatory center from just one single cone.
Now what does that mean? What I didn't emphasize before, but I'm sure you all know, that we have three major classes of cones, which some people call red, green, and blue. And then when you say that, the real aficionados say, come on, you can't say that. You're supposed to say short, medium, and long wavelength sensitive neurons. But we'll just call them red, green, and blue.
Now the reason, then, is that the center of one class of midget cells gets input only from red cones, another from green cones, and some from blue cones. I'll show that in more detail in a minute. So we have these three subtypes.
And so therefore, you can say that the midget cells, because they're so teeny, they should be able to see fine detail. And because of this wiring, they should be able to tell you about color. By contrast, if you look at the parasol cells, they get a mix of inputs, both in the center and their surround, so it's unlikely that they can tell you about color. And we'll examine that's in much more detail soon.
All right, so now the other interesting thing about this system is it makes you speculate as to why we have them-- and we'll elaborate on that-- is that the midget system, when you turn on the light, when you shine a small spot in the center receptive field, gives a fairly sustained response for the brief time that was maybe a second of two, OK? But the parasol system gives you only a burst, like that, instead of a sustained response. So think about that. Say why would we have two systems which are not only different in size, but also different in the profile of their responses? And then, once you have this question in your mind, then you'll be eager to find out when we talk about that time after next.
OK, so now let's go back to the beginning here, and talk about the photoreceptors in just a bit more detail, so you can get a better picture of it. Here, as I mentioned to you before, in the fovea, you have a very, very tightly packed cone photoreceptors. They're almost hexagonally shaped rather than round, they're so tightly packed.
But then when you go five degrees out, you first of all see that the cones got to be much larger. And now you have an intermixture of rods there that you can see. | in this region, you can process both information at low illumination levels and high illumination levels, whereas here as Keffer Hartline had proven, you can only see under fairly bright, normal illumination conditions.
OK, so now if you go even further out in the retina-- this is a beautiful, three-dimensional looking picture-- it shows the rods and the cones in the periphery. There are many, many more rods per unit area, and the cones have gotten even bigger. And so obviously, the ability for the cones to process fine information has been degraded as a result.
Now again, as I've mentioned to you before, we have in humans about 120 million rods and five million cones in each retina. OK, so now if you look at the overall distribution-- this is not too important-- you can see that as you go away from the fovea, there's a very rapid decline in the number of cones. This is the hash line.
And then there are no rods in the fovea, but they increase rapidly, and then they fall off. So that's sort of the overall distribution. And therefore, you can see fine detail in the center, and you're very sensitive for night vision not in the far periphery, but sort of in the mid section here, anywhere between 20 and 40 degrees from the fovea.
Let me remind you, because I'm sure you know all this already, a few facts, OK? I will just write down a couple of these things. First of all, what is degree of visual angle? That's a good question. What does it mean on the retinal surface?
Just let me give you an interesting mnemonic. If you stick out your arm like that, and you look at your thumbnail, that imprints one degree of visual angle on the retinal surface. OK? Now we are going to talk a lot about very small measurements. And so when we talk about small measurement, I mentioned already that the tip of a microelectrode is about one micron.
And what is a micron, anybody know? Yes.
AUDIENCE: 10 to the negative 6 [INAUDIBLE].
PROFESSOR: OK, one micron is one-thousandth of a millimeter, OK? Now, talking about that in terms of millimeters, which you're going to do a lot-- that's the greatest convention-- you need to also know what is the relationship between inches and millimeters, centimeters? OK, so if you take one inch-- you all know what an inch is, I guess. Roughly, if you look at your thumbnail again, it's maybe a little bit under an inch.
Now 1 inch equals 2.54 millimeters. So that's a very interesting mnemonic to remember. So whenever you look at various things and try to understand size, you may need to make that conversion, especially if you have always thought about things in terms of inches. Yes.
AUDIENCE: I think you meant to write centimeters.
PROFESSOR: What?
AUDIENCE: Did you mean to write centimeters?
PROFESSOR: You're right, 2.5 centimeters, 254 millimeters. You're right, OK. Sorry about that. Very good. Thank you for pointing that out.
OK, so now what we're going to do next is we're going to move on, and I'll tell you another clever experiment that had been done. These people here, McCrane et al., developed a method which enabled them to selectively label the blue cells in the retina. And this shows a picture of that. All the rest of them are red and green cells.
So only one out of eight cones is in the foveal area, near foveal area, I should say-- this is both the fovea and the perifovea is black. The rest of them are red and green cones, OK?
Now let's become specific about the facts here, and tell you first of all that one degree-- roughly one degree, which I pointed out is like a thumb, comprises 200 microns on the retinal surface. The intercone distance in the fovea is only 2.4 microns, OK? Whereas when you-- per square millimeter I already mentioned this-- you have 200,000 cones. You go out five degrees, it reduces tenfold. And then-- this I already pointed out to you several times. That's a good thing to remember.
And one thing I haven't mentioned before is that if you look at 12 font letter, I, and you look at it at 23 centimeters, that activates about 80 cones. So that gives you sort of a handle on what is the nature of activation there. And I already said that several times-- namely that each rod has 1,000 disks, and each disk in it has 10,000 molecules.
OK, and as I've just mentioned, one out of eight cones is blue. The others are fairly equal in number, but varies from person to person. OK, so now we are going to tell you about yet another experiment. And this is an incredibly clever experiment that was carried out by Ursula Drager.
At that time, when she did this beautiful experiment, she did this at Harvard, at the Harvard Medical School. And what she did was this-- she took the words Fiat Lux, put it on a screen, and exposed it that too dark to the animal-- the animal being a mouse, OK? And then her procedure enabled her to label, using a monoclonal antibody label, those cells that had been activated by Fiat Lux.
OK, so this is, then, the actual retina, flat-mount retina, showing you what had been activated. Now that's remarkable, beautiful, and she came, and gave a talk here at MIT maybe about 15 years ago. And when she presented this picture, one of the students, it wasn't any of you, I guess, raised her hand and was very upset-- said, but, but, but-- and so Ursula said, yes? So what do you think this listener asked?
Well, this was a very active thinker. This person said, but, but, but, was this image right-side up on the retinal surface? That was the question. And Ursula Drager said, no, it was upside down. I just rotated it 180 degrees so you can see it.
All right, well, interestingly enough, there were times when this became an issue. First of all, before people knew about lenses and that lenses turn images upside down, and secondly, because many people didn't believe that the images were upside down in the retina. I mean, if images are upside down in the retina, they're upside down on your brain, and yet the world is right-side up. What's going on?
So I would say about eight or ten years ago, I was asked to review a paper for a high visibility journal-- I can't name the journal-- in which the investigator very, very vociferously proclaimed that we have all been wrong, and that the images are right-side up on the retina after all. So what was his proof? His proof was that he took an ox eye, a slaughtered ox's eye, and cut out a little piece in the back of the eye, and put a translucent piece of paper over it, and then presented a stimulus out there. And when he looked at the back of the eye, at the translucent part, the image was right-side up-- a little bit blurred but right-side up.
And so he felt that he proved that images are right-side up on the retina after all. Well, I was flabbergasted. And it took me quite a while to figure out why this guy got what he got, because he'd obviously got what he got. So can you guys think of any reason for this? How come he got what he got?
AUDIENCE: Wait, what did he do?
PROFESSOR: He took the eye, OK, and in the back, he cut out an opening and put a transparency over it, so that anything came in there, you could see what would have been normally on the retinal surface. And it was a clever experiment-- no question about that. So what was wrong with the experiment?
Well, I'll tell you. What was wrong with the experiment was back to what I told you before, about the way the lens works, right? The lens works so that when you look at something close, the muscles of the lens are such that it makes the lens fat. And when the muscles relax, it makes the lens thin, OK?
So that means-- just to be graphic about it-- that if you have a thick lens, you have a short focal length. And if you have a thin lens, you have a much longer focal length, like that, OK? So now if you look at the back of the eye here, where the photoreceptors are, in this case, it looks like it would be upside down. But if they were in the same distance here, it would be blurred and it would remain right-side up, just like it is with a magnifying glass, OK?
So what this guy didn't know is the rule of how the muscle works in the eye to control the lens. And when the animal was killed, it no longer had the lens at the proper focal depth. And therefore, the image was right-side up.
So therefore, guess what happened? I wrote back to this high visibility journal saying that this guy is wrong for this reason. OK, so that was certainly an interesting experience, and that's why I managed to learn a little bit about how the lens works in the eye, which is a remarkable way it works, since it's so totally different from what we are familiar with when it comes to cameras.
OK, so now let's go to the next step, one down from your from photoreceptors. And we come to the so-called bipolar cells. Here's a series of pictures, the so-called [? cat ?] bipolar cells.
And what was discovered is that the bipolar cells here in the outer plexiform layer, they connect with the photoreceptors. And in the inner plexiform layer, their axonal projections are such that in the upper layer here, which is actually layer A, the cells there are OFF cells. And the ones that project below this here are the so-called ON cells. So there's a separation of the so-called ON and OFF cells at the bipolar cell level in the manner in which they connect.
And so therefore, those ganglion cells that connect with these are OFF ganglion cells. And those that connect with these are ON ganglion cells. All right, so that's basically the bipolar cells. And then we come to the horizontal cells.
Here's a beautiful example of a Procion yellow-labeled horizontal cell. And if that is then analyzed in detail, it looks something like this. This particular cell has 15 connections with the photoreceptors. And these cells-- I might as well anticipate-- we'll talk about that in more detail later-- they play a central role in providing the surround inhibition that I described to you for those retinal ganglion cells.
Then if we proceed to the amacrine cells-- and again, things are so complicated-- the numerous, numerous amacrine cells, different types-- and they have been studied extensively-- they are more than 20. And here's a clever experiment I want to point out to you. What you can do here, if you look at the called cholinergic amacrine cells-- that's one class-- you can selectively label them, because they're cholinergic.
And here's an example. All those lighter blue ones are your cholinergic cells. And then what you can do, you can put this under a microscope. And then you can lower a microelectrode into one of these cells, just like I've done here. And if you look at this closely-- this is not the best of pictures-- this is a Procion yellow-labeled amacrine cell-- you can see it has all these processes.
So then if you look at this on the microscope under the high quality, you can draw these out. And this is what they look like, OK? So this is a cholinergic cell.
This is another type. It's just called A2, which has a lot to do with rods. And then yet another class called open [? minergic ?]. I'm not going to go into detail about it. But you can see that each of these now-- scientific methods enable you to specify what these amacrine cells are like. And on the basis of that, people have come up with ideas what the amacrine system does.
OK, now we come to the crux of things in the last few minutes. We're going to talk about the electrical responses in the retina. Now this is going to be, again, fairly complicated.
This work-- again remarkable work which, in my view, merits a Nobel Prize, but it has not yet been awarded to John Darling at Harvard. And let me just introduce this by telling you how they went about this experiment. They realized that the cells in the retina are very, very small. And so it's very difficult to record from them intercellularly.
You have to do that if you want to be able to label them anatomically. And so they looked around for a species of animal that had large cells in the retina. And they discovered after a lot of search that the so-called mudpuppy-- Necturus maculosus-- is an animal that has unusually large retinal ganglion cells.
Then they developed a technique of removing the eye from this animal, and putting it into a dish, because that makes it real stable. And then they were able to put an electrode in there, define what the cells did functionally by shining light on them and looking at the activity, and then they were able to label the cell by injecting a labeling substance. So that's what they did.
And so here is a description of that arrangement. Here you have an inverted mudpuppy retina, and here you have a DC-recording electrode. And what you do then, you look at it in an oscilloscope. And at this point, the cell is entered by the DC recording. And then once it's inside, cells inside are negatively charged with respect to the surround, usually up to about 70 millivolts. In this case, I have 50 millivolts labeled.
And so once you're inside the cell, you see a sudden drop to a minus level. And then if the cell discharges an action potential, then you see that, of course, in the oscilloscope. And then you can study this cell in all kinds of detail as to how it responds, what it responds and so on, to determine what its characteristics are.
So then when they did that, they made the following central discoveries-- they discovered, first of all, that receptors all hyperpolarize to light. Now this is the opposite of what you would have thought, because the principle of the way cells operate-- and I'm sure you know all this, but I will also describe it in a bit more detail in a minute-- cells when they hyperpolarize, they are less likely to cause a release in neurotransmitters. If they depolarize, they will increase the likelihood of neurotransmitters being released.
And by the way, the neurotransmitter for these receptors is glutamate. And also, which I should emphasize here, is that the receptors never give action potentials. They only give graded potentials. That's also true for the horizontal cells and the bipolar cells.
They found that all of receptors hyperpolarize to light, meaning that they activate subsequent elements in the retina when it gets darker out there, rather than when it gets lighter, which is the opposite of what we all would've expected. Same thing for the horizontal cells. But then when you come to bipolar cells, suddenly you find that there are two different classes-- one class which mimics what the receptors do, the other class that does the opposite. And so the bipolar cells of these two types-- this type has sign conserving synapses, and this type has sign inverting synapses.
Now amacrine cells come in various types, and some give action potentials. And ganglion cells all give action potentials. So to go through this again, all receptors hyperpolarize to light. Horizontal cells hyperpolarize to light. About half of bipolar cells hyperpolarize and half depolarize.
Now this is accomplished-- by the way, I'll go into that in more detail later-- by virtue of having different kinds of neurotransmitter receptor sites. And I'll talk about that in a lot of detail when we talk about the ON and OFF cells. OK, and then we have the amacrine cells. Some hyperpolarize, some depolarize, and some give action potentials. And all ganglion cells give action potentials.
So that is a major, major discovery, and not only major, but totally unexpected from what one would have thought. All right, so now let me just explain this in a bit more detail. If you go inside a cell, then if this cell is activated, it can be either get-- what you see is an excitatory post synaptic potential, which goes from minus towards plus, or you can get an IPSP, an inhibitory post synaptic potential. And this discovery was made by Eccles, which is a remarkable discovery for which he received the Nobel Prize some years back.
All right, so now if we look at those cells which give action potentials, what happens is when you get an EPSP-- that's why it's called excitatory post synaptic potential-- then you increase the likelihood that an action potential will be generated that will send its signal down the axon to the next location of the cells. All right, so that's the basic process.
So again to reiterate, photoreceptors hyperpolarize the light. Therefore, glutamate is released when there's a decrease in illumination-- the opposite of what anyone would have expected. All right, so that I think brings us to what I want to cover today. I'll wait until next time to talk about the lateral geniculate nucleus, which is very brief.
But let me go through the four receptor basics again. All photoreceptors hyperpolarize the light. Depolarization of the photoreceptor releases glutamate. Photon absorption by the photo pigment results in isomerization of the chromophore from 11 cis to 11 trans. This is what I told you to think about in a very simple fashion-- to say that the photoreceptor molecules-- rhodopsin in this case-- is either bleached or unbleached, OK?
So once it's bleached, further light has no effect on it. And at any point, given whatever the level of illumination is, a certain percentage of these rhodopsin molecules are bleached and a certain percentage is unbleached. The darker it is, the more are unbleached.
All right, then we have these two classes that we talked about-- the ON and the OFF cells that we will discuss in some detail-- the synaptic junction of OFF bipolars is sign conserving, as I just said, and that of ON bipolars is sign inverting. Now the ON bipolar receptor is-- I should mention that now, and I will deal with it in detail-- is called the mGluR6. It's activation leads to closing of channels causing hyperpolarization.
that's the basic layout, then, of the photoreceptors. And I will now stop at this point. And next time, we'll talk a little bit about the lateral geniculate nucleus. And then we're going to move on to talk about the cortex.
then if you look at you assignment sheet, you can see here that on September 16th, we're going to talk about the ON and OFF channels. And then we'll deal in more detail with these rather complex things which are pretty hard to remember, about hyperpolarization, depolarization, glutamate, glutamate receptor sites, and so on. OK, thank you.
Free Downloads
Video
- iTunes U (MP4 - 180MB)
- Internet Archive (MP4 - 180MB)
Subtitle
- English - US (SRT)