Flash and JavaScript are required for this feature.
Download the video from iTunes U or the Internet Archive.
Description: This lecture covers cochlear anatomy and vibration patterns. Topics include the inner and outer hair cells, OHC electromotility and otoacoustic emissions.
Instructor: Chris Brown
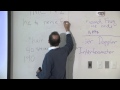
Lec 15: Hair cells
The following content is provided under a Creative Commons license. Your support will help MIT OpenCourseWare continue to offer high quality educational resources for free. To make a donation or to view additional materials from hundreds of MIT courses, visit MIT OpenCourseWare at ocw.mit.edu.
PROFESSOR: Maybe I should go over real quickly what we did on Monday. We can start out with we covered the various parts of the auditory periphery-- the external, middle, and inner ears. And we had a paper on the function of the external ear and how it can help you localize sounds when you distort it by using clay molds that you can put in subjects' pinnae. Your sense of localization of sounds, especially those at different elevation, is all screwed up.
And you can relearn because you still have some bends and quirky geometry in your pinnae. Even with a little clay in there, you can relearn using new clues to localize sounds in elevation, but it takes a little bit of time. So the subjects in that paper relearned how to localize sounds.
Now, there are other cues that we'll be dealing with for localization of sounds in azimuth. And that's where you use two separate ears. For example, if a sound's off to my right, it's going to hit my right ear first. And then because sound travels at a finite velocity in air, it's going to hit my left ear second. So there's a delay between the two ears, and that's a primary cue for localizing in azimuth, in the horizontal plane.
So one comment I had after class was that was a good paper on relearning sound localization with new ears. Someone came up and said they had read that in another discussion group. And I think it is a very good paper, because it deals with the function of the external ear. It deals with how you can relearn new cues. Let's say if when you grow up, your pinnae are getting bigger from being an infant to an adult. So you have to relearn those cues over time.
We talked about the function of the middle ear, securing efficient transmission of sound energy from air into the fluid of the inner ear. And we talked about, of course, the physical characteristics of sound, what sound frequency is, what sound level is. We talked about simple sounds-- that is, pure tones or single frequencies. More complex sounds like musical sounds that have a bunch of frequencies, and even speech sounds that have a whole bunch of different frequencies and they're changed a little bit. And the change is very perceptually obvious in the form of changing from one vowel to another vowel.
And so another comment I got by email, someone said, well, can you tell me where you're from? Because you have a Midwestern accent. OK, speaking of speech sounds. And that's a very interesting comment, because I've been in the Boston area since 1983. And that's 30 years. And I am from the Midwest. In fact, I brought my Midwestern clothes today. I got my hat and my Michigan sweatshirt which I got out of the rag pile. Or is it my Harvard sweatshirt? Let's see. It says, "Harvard, Michigan of the East." So I guess it's my Michigan sweatshirt. It's got the Michigan colors, maize and blue.
And so I grew up in Ann Arbor, Michigan. I probably-- it depends on who you are, you have to sort of listen for it. According to my wife I still have it, and maybe some of you can hear my Midwestern twang. So it's an evidence that you have very good hearing that you can hear these different not only vowels but accents.
So that's kind of interesting that I was reading a book just last week. And it's called Our Boston. You can get at the MIT COOP. And one of the chapters is on regional accents. And I'll do a little reading maybe just at the very beginning of this chapter which talks about regional accents and linguists. I'll just read you the first sentence.
It says, "As most linguists might tell you, regional accents are a lot like underpants. Everybody has them, and usually no one notices his or her own. But the world would be a very different place in their absence." So that's what regional accents are. How many of you guys are linguists, or interested in linguistics? Just a few, OK. Well, of course is it's a fascinating science, and it intersects with the auditory world.
So today we're going to launch into the next aspect of the auditory periphery which is the inner ear. And the inner ear, the scientific name, of course, is the cochlea, from the Greek snail shell. We're going to talk about the anatomy of the cochlea. This is the cochlea here.
We're going to talk about the vibration patterns in the cochlea. Because of course, the cochlear structures are vibrating in response to sound. And the original pioneer in that area was of course George von Bekesy, who was a Hungarian physicist who worked for the telephone company, later came to Harvard and won the Nobel Prize in 1961. So we always put him up on the pedestal because he is the only winner for the hearing field for the Nobel Prize.
So those in the vision system, you can pull out all these people who've won the Nobel Prize. In the auditory field, there's one winner. It's George von Bekesy. So we'll talk about his work on cochlear vibration patterns.
Then we'll talk about the receptor cells for hearing, which are inner hair cells and outer hair cells. There are two types, a little bit like you had the rods and cones in the retina. But these have very different and complementary roles, and we'll talk about the separate functions of those two types of hair cells.
We'll talk about the outer hair cell electromotility. We'll go into what that is. And finally if we have time, we'll end up with otoacoustic emissions, which are a very interesting research and clinical tool for testing here.
So cochlear anatomy, here is an early drawing of the cochlea by DeVerny. Back in the 1600s, they already knew that the cochlea was like a snail shell. And in the middle of it, it had a membrane, and the membrane went all the way from the base to the apex. This very basalmost part is called the hook. And then the membrane spirals around and goes to the very apex.
And so I have a very simple wire model that I can hold up, do the same thing. I can bend it any way I want to. Here's the hook. You can see this in three dimensions here. OK, so the cochlea is a spiral shaped structure which has a membrane in it.
The name of the big membrane-- it has several membranes-- the name of the big membrane is called the basilar membrane, which of course stands for base. And we'll see that the hair cells and the other cells associated with them sit right on top of the basilar membrane. We can go to that in just a second.
Anatomists more recently like to cut sections through structures. And that's because the light microscope, the electron microscope, can resolve very small things like cells and parts of cells very well in thin sections. So if you cut right down the middle of that snail shell and took out a thin section, you'd get this view.
So of course, all this gray shading on the outside and even parts of the inside is bone. So this is a bony structure. It's very different from the eyeball, which is all soft tissue. This is a bony structure. In the middle, there is a nerve coming in. That's the auditory nerve. The auditory nerve starts in the cochlea and sends messages to the brain, which is down here. It's been cut away.
You can see the tube of the snail shell, or cochlea, is subdivided by this big membrane, this basilar membrane. And that there's another membrane in here too. The name of that membrane is Reissner's membrane, but it's not that important. But the picture you get here sort of looks like steps on a ladder. And the Latin name first steps is "scala"-- or "scalae," if it's plural. This means steps. For instance, a musical scale is whole steps, and every now and then a half step.
So these, to the early anatomists, looked like steps on a ladder. But actually what they are, are fluid-filled compartments separated by membranes. And there are three fluid-filled compartments here. And this is another cross section of just one turn of the cochlea. This compartment is called scala tympani. This compartment is called scala vestibuli. And this one, which is in the middle, is called scala media. So there are three scalae in the cochlea. So scala tympani, vestibuli, and media, or middle.
And the hair cells sit on this membrane that separates scala tympani from scala media. And the hair cells here, the inner hair cells and the outer hair cells, are surrounded by a whole bunch of other cells. And that whole receptor organ is called the organ of Corti. So it says that down here, the organ of Corti. And Corti was one of the first Italian anatomists to really draw the structure correctly, although he got it a little bit wrong.
These round circles are the outer hair cells. If you cut this in cross section and look at this in a magnified version, there's only one inner hair cell. He put two in his drawing, so it's a little bit incorrect. But his name is now attached to the organ. The organ of Corti is where the hair cells are, and there's a whole bunch of other supporting cells that keep the hair cells in their place.
OK, so the receptor organ sits on the basilar membrane. And the organ of Corti's there. There's this other membrane that separates scala media from scala vestibuli. It's called Reissner's membrane, but it's not important. OK, so that's the structure of the organ of Corti.
And during the 1930s and '40s, people started thinking about, well, how does this thing move when you stimulate the ear with sound? And George von Bekesy, as I've said before, was the first to make really good measurements of the motion of the basilar membrane in response to sound. And this is a cutaway diagram of his experimental setup.
This is the cochlea in cross section. Of course, he had the whole cochlea there. And he used cochleas from cadavers. He would go to the mortuary, get a temporal bone and drill away all the bone and be left with the cochlea and put it in his Petri dish. He had a huge sound source that he would apply right to the oval window. He would take out the stapes and drive the cochlear fluids directly.
And as we said last time, if you have sound in air and are trying to get it in fluid, you really to crank it up. And so it's a huge sound source. And he stimulated way up at 140 dB, way at the top end of the curve we had last time. He needed to drive it that high not only to get sound into the fluid but also so that his measurement system could pick up these movements. The movements tend to be very, very small.
In response to sound, maybe the basilar membrane is moving only a few nanometers. OK, but it's going to move a lot more nanometers if you blast the heck out of it. And his observation device was simply a microscope, a water immersion lens that he brought down and focused on the basilar membrane to see the thing move. So he didn't have very good resolution. Maybe he could only see micrometers in terms of movements, so he had to turn up the sound very high to get the thing to vibrate.
So we can criticize his experiments now for several reasons. Number one, we don't listen way up at 140 dB. In fact, those kinds of sounds are actually damaging to the hair cells. But we can also say there weren't any hair cells. It's a dead preparation. It's a cochlea from a cadaver, OK?
And what we know now is that the movements and vibration patterns of the cochlea are very different in a dead preparation than they are in a living preparation. We'll get to that in just a minute. In spite of those problems-- I mean, von Bekesy was working in the '30s. He didn't have very good measurement devices, he had to use what he had. He discovered some very important things.
One of the things was that the basilar membrane-- and here's a diagram of the basilar membrane. It's this horizontal line here, if there's no sound. And it's stretched out now as if you took the snail shell and unwound the coil and made it a long, straight basilar membrane. So the base is over here and the apex is up here.
And we think that that doesn't change the vibration pattern at all. The only reason that the cochlea is coiled is to save space in your head. Otherwise, it would be pretty long. So unwinding this is just a convenient way to look at the vibration pattern. He measured in the intact cochlea, of course.
This pattern of vibration that von Bekesy observed was called a traveling wave. OK, so this-- traveling waves in the cochlea. And basically what that means is these are snapshots-- one, two, three, and four. At one instant, the wave pattern looks like number one. At the next instant, it moves, or travels, and looks like number two, and so on and so forth, three and four.
So the traveling wave starts in the base and travels up to the apex. And remember, the base of the cochlear is where the input is coming. If we go back to our first diagram here, remember, the stapes is pushing in and out at the oval window. And that's way down at the base of the cochlear. And what we're seeing is these vibrations are traveling from the base all the way up to the apex, and it's taking a little bit of time to do so. So that's what von Bekesy discovered-- there's a traveling wave.
The second thing he discovered is that the peak of the traveling wave-- you notice these dash curves draw the envelope or the maximal point of movement of this basilar membrane. There's a peak of this traveling when the basilar membrane is vibrating the most. That peak changes as a function of the frequency of the sound that you used to stimulate your preparation.
And this diagram shows that, but I have a little better movie, or demonstration, that shows you a traveling wave that's a little bit easier to understand. So I actually have two of them. These were made by Chris Shera at Mass Eye and Ear Infirmary. And again, this is the basilar membrane stretched out in a long line from the base of the cochlea up to the apex of the cochlear. And this is a situation with no sound.
So now we're going to turn on a sound that is a low frequency tone. So tone is synonymous with pure tone or a single frequency. So it's just going to be one frequency. And the input comes in here at the stapes. And you'll see the pattern of movement of the basilar membrane in response to the low frequency tone. Whoops, pressed the wrong button.
It takes a little bit of time for this to develop. And then you can see the complex pattern, or traveling wave. It seems to start here and go up to here. It goes up to a peak and then quickly comes down. There's almost no movement right at the apex there.
So one thing about that movement that I said before is there's a peak of vibration at a certain place along the basilar membrane. The other thing is that at any point along the basilar membrane that's moving, it's moving as a function of time. And actually, if you knew what the frequency of that tone is and looked at the frequency of movement here, it would be the same. So the basilar membrane is moving up and down at the same frequency as the sound stimulus.
Maybe that'll be a little bit more clear when I go to the next one, because the next movie shows the basilar membrane vibration to three tones-- the low one that we just saw, a middle frequency one, and a high frequency one. And as you can indicate, you can probably get from my hand movements, the low frequency tone is going to maximally vibrate here toward the apex. The middle frequency is going to be maximally vibrating here, and the high frequency is going to be maximally vibrating over here. OK, so there's going to sort of a frequency analysis along the length of this basilar membrane.
The other thing I want you to notice when the movie is playing is how fast these three places along the basilar membrane are moving, see if they're moving at the same speed or at a different speed. So this is the one we had before. This is the middle frequency and this is the high frequency. See how much faster this is going back and forth than the slow one, and see that it's peeking in a different place?
OK. So this is, we think, due to the physical characteristics of the way this membrane is set up. For example, down here the membrane is fairly tense and it's very short in width. It's like those little tiny strings on the piano way up at the right end of the piano for the high notes. And it's naturally going to vibrate at high frequencies.
When you get up toward the apex, everything gets real wide in terms of the membranes. The cells get bigger. Everything's heavier, and it's naturally going to vibrate slower. And it's going to vibrate best for low frequencies. OK, so there's this analysis along the length of the cochlea in terms of high frequencies are mapped here, mid frequencies are mapped here, and low frequencies are mapped here. There is, if you will, a place code for sound frequency.
So sound frequencies are broken up into a place code-- low frequencies apically, and higher and higher frequencies basally. Secondly, if you buy into my frequency, or our timing of the movement, there's also a timing code in that high frequencies are vibrating much quicker and low frequencies are vibrating much slower.
And as we'll learn in a week or two, the nervous system keeps track of both of those things. There are hair cells down here in the base of your cochlea which are connected to the nerve fibers that only innervate them and are only active when there's a high frequency sound stimulus. They send that message to the brain. The brain says, ah, here are some high frequency or high pitched sounds. Conversely, there are hair cells up here that are only responding when the basilar membrane is stimulated with a low frequency. And they're telling their nerve fibers only send action potentials to the brain when there's a low frequency sound stimulus.
So what are examples? Everybody knows what a low pitched and a high pitched sound is. But for example, one of the ways you tell a male voice from a female voice is that female voices have higher pitches to them, higher frequencies, maybe an octave higher. So immediately usually, even over the telephone, you can tell I'm talking to a female speaker because the frequencies are higher and they're mapped more toward the basal end of your cochlea. Male voices that have lower frequencies are mapped to more apical positions. Any questions about that?
Now how do we, in the modern times, well beyond von Bekesy, how do we measure these motions? You're sort of taking it for granted from me that we can make these measurements. Well, von Bekesy used an ordinary light microscope. And he really had to crank things way up to see the structures moving. And we want to measure them down near where we usually hear, 20 dB SPL, 40 dB SPL.
So what's used is, on to the basilar membrane, you put some sort of source. And maybe in the 1980s and '90s, people were using radioactive sources and using the Mossbauer technique. They knew that the radioactive particles being emitted had a certain frequency, and when the basilar membrane was moving toward you, the frequency would be changed versus moving away from you.
Nowadays in the '90s and 200s, people are using spots that they put down, like little reflective disks that are put down onto the basilar membrane that reflect light. And you shine down a light onto the basilar membrane. And that spot reflects the light back to you. You can compare the light you sent down with the light that's reflected back.
If the membrane is moving away from you, that light will be shifted to a higher wavelength versus if it's coming toward you, it'll be a lower wavelength. And this process is called interferometry. And if the source is moving like the basilar membrane is moving when you stimulate it with sound, you can use a laser to shine the light, and you can use the Doppler interferometer-- which is a device that measures the shift in light when there's a moving light source or a moving light reflection.
You can calibrate this in terms of the displacement or the velocity that the object doing the reflecting is giving. It's shifting the light, basically. So that's how most modern experiment experiments measure of the movement of structures, even though they're very, very small movements, like in terms of nanometers.
So let's see what this-- these are data from the 1980s using a Mossbauer technique, a radioactive source. So instead of reflecting light, you're measuring the emitted wavelengths of particles from a radioactive source at one point on the basilar membrane. And you're stimulating the basilar membrane with sound of different frequencies, and you're measuring how much it moves in terms of the basilar membrane. I think this says displacement. Is that what it says? It says amplitude in dB.
This is a displacement scale, how much movement you're getting, as a function of sound frequency for one particular point on the basilar membrane. So you change your sound frequency. Start with low frequencies at 20 dB. Pretty low level sound. You don't find much displacement. Go up to 6 kilohertz, find a little bit more movement. Go up to 10 kilohertz, it's getting bigger.
And then all of a sudden at about 16 kilohertz, you get a huge amount of movement. You're right at the peak of the traveling wave. Go up to 18 kilohertz and it goes way back down again. And 20 kilohertz there's no point plotted because you can't get the thing to move at all. It's a very sharply-tuned function of movement in terms of the sound frequencies. This place is only moving-- or mostly moving-- for a sound frequency of 16 kilohertz in terms of where the source was.
Change the sound level to 40 or 60 or even 80 dB-- 80 dB is a sound that is certainly within conversational speech. It's a high level sound, but it's not painful or damaging at all. In that case, the function is much broader.
If you just looked at that function, you'd say, well, this point on the basilar membrane years is responding to many sound frequencies. It's not responding to 20 kilohertz, but it's responding to everything below that. And if you were to-- they didn't here because they wanted to take care of their preparation and not damage it-- if they want up to the levels that von Bekesy used, 140 dB, it would be completely flat.
And von Bekesy found that the tuning of the basilar membrane was very flat, or just broadly tuned. But these modern measurements show extremely sharp functions for a single point of movement on the basilar membrane. This is a plot of the same data, but plotted a little bit differently. This axis is still sound frequency.
And now we're going to plot it on the x-axis. It's still a dB scale, but now on the y-axis it's threshold dB SPL. So what's threshold? Well in this case, it doesn't really make sense. I think it would've been better to label this some criterion displacement, because the criterion displacement use for this lowest curve is 0.35 nanometers.
And what the experiment now, or what the plotting now is how much sound level do we have to crank into the system to get this point on the basilar membrane to vibrate 0.35 nanometers? OK, in the case of 16 kilohertz right here, at the lowest point, we only had to put in 10 dB, not very much sound at all. At 14 kilohertz, at 19 kilohertz, we had to turn the sound up a little bit.
At 20 kilohertz, we turned up the sound so much, but we said could never get it to vibrate 0.35 nanometers. So there's no point plotted there. At 5 kilohertz, we had to crank up the sound to about 60 dB to get this point to vibrate 0.35 nanometers. So you're asking for the point to give you some specified amount of vibration or a criterion of vibration. And you're plotting this function here.
And this is a very important curve. We'll be seeing this many, many times the rest of the semester. And it's called a tuning curve. So you should all be familiar with how this tuning curve is generated. You can make a tuning curve for a recording from a hair cell. You could make a tuning curve for recording from a nerve fiber.
Let's say we put on an electrode in the auditory nerve. You guys have talked about this term, this technique called single unit recording where you put an electrode in a nerve-- say, the optic nerve-- and you measure the spikes coming from the single axon that you're recording from. And you might say, well, I'm going to turn the sound up until I get 10 spikes per second. That's my criterion.
And I'm going to change the frequency. How much sound level do I have to stimulate the ear with to get the auditory nerve fiber to fire 10 spikes per second? Well, at 16 kilohertz I have to hardly put any sound in at all. But 2 kilohertz, I have to really blast the thing. So this auditory nerve fiber is very tuned to the sound frequency. It's sharply tuned, OK, to a frequency near 16 kilohertz.
You could make a tuning curve from a receptor cell by saying how much receptor potential do I want? Let's say one microvolt of response or receptor potential from the hair cell. What sort of sound level do I need to dial into the ear at these different frequencies to get that criterion receptor potential? Is that clear?
So these tuning curves are omnipresent throughout the auditory pathway. And they're a measure of the sharpness of tuning. They're a measure of whether one place on the basilar membrane or one auditory nerve fiber can listen to 16 kilohertz and ignore 20 kilohertz. It's very important if you're trying to know in the pattern of harmonics that harmonic number two is missing, you better listen to number two with a very sensitive and sharply-tuned function. And you have that in the auditory system starting with the vibration of the basilar membrane.
What are these receptor cells for hearing? So the receptor cells for hearing are called hair cells. That's kind of a funny name, right? But they get their name from the fact that they have hairs coming out the top of them-- at least, it looked that way to the early neuroanatomists. So these hairs are more properly called stereocilia. And let me write that down here, stereocilia.
And even that more proper term is kind of a misnomer, because it has as part of it "cilium." That's really a misnomer. What is a cilium on a cell? Does anybody know?
AUDIENCE: [INAUDIBLE].
PROFESSOR: Yeah. And they can propel single cells through a medium, right? And they're wavy and floppy, and if you look at them in cross-section of the electron microscope, they have these sort of 9 plus 2 tubular arrangements. The stereociliae do not look like that at all. They should be called stereomicrovilli, because when you look at them in cross section you see all these filaments but there's no organization to them.
And you can see the filaments right here in longitudinal section going up into the stereocilia. And these keep the stereocilia very stiff. So when sound comes and moves a stereocilium, it's sort of like a telephone pole in a hurricane. It pivots around its base, but it doesn't flop around. And the base of these stereocilia are right as they insert part of the cell right. They're very stiff structures.
Furthermore, they're all attached one to another. So all the stereocilia on a hair cell tend to move together back and forth in response to sound. And especially, they're attached, of course, at the base, but they're also attached at the very tips in something called tip links. And I think I have a picture or a diagram. So here are some tip links that connects the very tallest part of one stereocilium to its next tallest neighbor stereocilium.
So the whole bundle of stereocilia move together in a rigid, pivoting way. And the whole bundle is usually called just in jargon terms, the "hair bundle" at the top of a hair cell. And I have some numbers for you. How many stereocilia are there in the hair bundle of a given cell?
So there are 40 stereocilia for one inner hair cell. We haven't talked about what these are, but these are in your cochlea. They have inner hair cells and outer hair cells. And there are about 140 stereocilia in the hair bundle of a given outer hair cell. OK, there are many stereocilia per cell.
In this cross section, you're just seeing three rows, but there are many per row. And here's a whole big hair bundle all together. Now, why are these exact numbers important? Well, it isn't that important, except we think that the channel that opens up when this hair bundle moves in response to sound is located right at the tips of the stereocilia. And most people believe that there is one channel at the tip of each stereocilium which would suggest that each outer hair cell has 140 channels and each inner hair cell has 40 channels.
Now, what are these channels? Sometimes they're called the transduction channels. What's transduction? Anybody? What's a transducer in engineering terms? Anybody? What does it mean to transduce something? Well, you can have-- yeah?
AUDIENCE: [INAUDIBLE]?
PROFESSOR: Exactly right. So you can have an accelerometer which converts acceleration to an electrical signal. In this case, the physical energy of sound is mechanical energy. It's movement, movement of the tympanic membrane, movement of the ossicles, movement of the basilar membrane up and down. These cells are sitting on the basilar membrane. And the hair bundle is moving back and forth.
But the code of the nervous system-- we've been talking about spikes-- somehow you have to get that mechanical energy into the electrical energy of, in the case of hair cells, the receptor potentials, and in the case of the nerve fibers, the action potentials that are going to send messages to the brain. So the transduction channel is the channel that responds to mechanical energy and allows ions to flow into the hair cell-- that is, it opens up.
And in the case of these transduction channels, it allows positive ions, mostly potassium because there is a high concentration of potassium in the fluid of scala media. Remember, these cells are sitting in between scala tympani and scala media. So scala media is up here. The transduction channel opens. And it's relatively non-selective, but the big positive-- it's non-selected for positive ions or cations. The big concentration here is potassium, so potassium is probably the ion that flows into the hair cells when the transduction channels open up.
And the evidence for that here is in part from Hudspeth's lab. He probed around the hair cells while the hair bundle was moved back and forth. And he found big potentials up near the tips of the stereocilia and very small potentials in the rest of the cell. So there's very good evidence that the transduction channels are at the tips of the hair cells.
We don't know what the transduction channels are. People are actively working on that. So there's a guy at Harvard in the Department of Neurobiology, Jeff Holt, who thinks the transduction channel, this so-called transmembrane channel, TMC, of which there are two varieties, 1 and 2. It's not clear if that's actually the channel or something associated with the channel. But when you knock it out, the hair cells don't respond anymore. The animal is deaf. OK, so these transmembrane channels may be the transduction channel, but the jury is still out.
OK, so let me go back to our generic hair cell. We've talked about the stereocilia here and their movement. That's the input end of the hair cell. The middle part of the hair cell has a nucleus. It has a membrane. It has mitochondria. And down here, you might think of this part as the output end of the hair cell. This is where the hair cell is giving its information to the associated nerve fibers.
And there are, interestingly, two types of nerve fibers. The one you would instantly think of is the afferent nerve fiber, the auditory nerve fiber, where the hair cell is sending messages to the nerve fiber. And how does one cell send a message to another in the nervous system? Well, there's a synapse, right?
So there's a hair cell to nerve fiber synapse. And that's right here. And this hair cell then releases transmitter, because this is a chemical synapse. And it uses a neurotransmitter. Right, everybody knows what neurotransmitters are. The neurotransmitter here is probably glutamate. And so that's an excitatory neurotransmitter.
OK, so the hair cell then releases the glutamate. It goes through the synaptic cleft. There's a glutamate receptor that it binds to on the auditory nerve fiber. The auditory nerve fiber is depolarized or excited. It starts to fire action potentials. And then these action potentials are then sent down the auditory nerve and into the brain. The brain says, aha, there's a sound.
Now interestingly, there's another kind of nerve fiber associated with many hair cells, and it's called an efferent nerve fiber. So what's the difference between an afferent and an efferent? anybody?
AUDIENCE: Directionality of the cell?
PROFESSOR: That's right. So, which one goes which way. We've said this one is going-- the afferent is going that way. The efferent nerve ending is going the opposite way. And so most of this naming nomenclature comes from the reference being the brain. The brain is where the action is, right? The cochlea's way out here.
So anything that's going out from the brain is efflux or efferent, so signals that are going from the brain out to peripheral structures-- like the hair cells-- travel by way of efferent nerve fibers, right? Another efferent type of nerve fiber would be a motor neuron, a motor neuron sending messages from the brain out to the periphery to contract the muscles. That'd be another type of efferent nerve fiber.
So interestingly, the hair cells have efferent nerve fibers attached to them, and they form synapses on the hair cells. And you do not see this in the visual system. You do not see efferent innervation of the receptor cells or of the retina at all in vision, at least in mammalian systems. You do see it in lower vertebrates.
But in mammalian systems, the auditory hair cells get an efferent innervation. There are hair cells as another part of the inner ear. We talked about the vestibular system and the semicircular canals. That is a hair cell organ, and those get efferent nerve endings from the brain.
And in the sides of the body in amphibians and fish, there's a lateral line system of hair cells that allows the fish to detect currents of motion of water. And those are hair cell-based receptor organs, and they get an efferent as well as an afferent innervation. So it seems like wherever there are hair cell-based systems, the brain sends messages out to the hair cells as well as getting information from the hair cells.
So we'll have a lecture later on what these efferent nerves are doing. I mean, why would the brain want to control the auditory periphery? It's an interesting question, and maybe there are several answers to that. Certainly, the brain wants to know what's happening to the hair cells. So these afferent nerve fibers are sending messages when the hair cell is stimulated. So that's the main pathway going into the brain.
OK, we've talked about the transduction channels. We haven't talked about these little things called tip links. One idea is that these links between the tips of the stereocilia are sort of like a rope on a trap door. And when the stereocilium moves, it opens up the trap door, and that's what opens the ion channel.
These little tip links are something you see in the electron microscope. They're very, very fine. When you use some chemical treatment to dissolve those tip links, the hair cells don't work anymore. They don't respond to sound anymore. So there are some other proteins up there associated with the transduction channels called tip links. That's a very active area of research now-- what are the transduction channels? How do the tip links work? How are they mechanosensitive?
OK, now let's talk. We've been alluding to the two types of hair cells, right? Outer and inner hair cells. And all mammals have these two types of hair cells, outer and inner hair cells. Birds have hair cells that are also of several classes. They have what are called tall and short hair cells. They're a little bit different than outer and inner hair cells.
Reptiles generally have one type of hair cell. So the inner and outer hair cell distinction is true for mammals mainly. So how do they get their names? Well, if you look down on the top of the organ of Corti-- so this is my wire model. So this is like the cochlea we've been looking at. Now, turn it so you're looking down from the apex down.
On top of this membrane, what you would see if you looked at one little tiny piece is a row of inner hair cells going from the extreme base to the apex, which would be this row right here. And three rows of outer hair cells, going from the base to the apex, and it turns out that the inner hair cells get their name because they're on the inner side of the spiral. And the outers are on the outer part of the spiral.
So the inners are toward the center, or toward the axis of the cochlea. The outers are away from it. And this view, looking down on them, would be looking down onto their stereocilia. And these white structures are the hair bundles-- the tips, if you will-- of the stereocilia. And there's one, two, three, four, five, six, seven, eight and a half inner hair cells here.
And in the first row of outer hair cells, there's one, two, three, four, five, six, seven, eight, nine, ten, eleven, twelve. There's a dozen outer hair cells. And their stereocilia are lined up differently. They're sort of like inverted V's on the outer hair cells. And there's the first row, the second row, and the outermost row, the third row of outer hair cells.
And this is a stereotyped pattern. Almost all mammals have this. In the human, if you go up into the apex of the cochlea, sometimes a fourth row of outer hair cells starts to form, but it's not very well organized. It's sort of patchy. But you can have a fourth row of outer hair cells.
If one of these were missing, the supporting cells nearby form a little scar in its place. And we'll talk about things that kill hair cells in a few weeks. Loud sounds can kill your hair cells. Infectious agents-- meningitis, for example-- can kill your hair cells. Certain drugs, aminoglycoside antibiotics like kanamycin can kill your hair cells.
And the supporting cells thereby just fill in. And you can go and look at a cochlea that has some hair cell damage. You can count, you can see how regular the array is of hair cells. And you can count how many are present there and how many are damaged. And you can, you know, say if there's a 50% loss of outer hair cells in row one, this regularity is so beautiful.
Unfortunately, once they're killed in a mammal, they never come back. You cannot regrow your hair cells once they've been killed. In birds, they grow right back. Takes about three or four weeks. So you can kill all the hair cells in a bird cochlea and come back three or four weeks later, and they're all grown back.
In mammals, they don't grow back. And so there's a lot of interest-- Because you have these agents that kill hair cells-- there's a lot of interest in, how can we make our hair cells grow back after they've been killed? And people are thinking of stem cell approached or neurotropic drugs that might be good. So why don't they grow back? We don't know that at all.
We do know that, for example, I'm in a department called ENT-- Ear, Nose, and Throat. Lots of the surgeons in our department deal with cancers of the head and neck, right? Because there are cancers that can come up, and surgeons can take care of that by taking cancers out. There are no known cancers that grow in the inner ear. It never, ever happens.
So maybe these cells are so far differentiated and they've become this classic inner and outer hair distinction, they're so evolved that they can't grow back. They can't multiply. They can't form a cancer. But unfortunately, once there destroyed by some agent like a drug, they can't grow back. So a way to put new cells in there that could become new hair cells, or a way to encourage these supporting cells on the sides to grow and become hair cells, is a very interesting idea, but one that's just in the research phase now.
OK, so that's the difference between inner and outer hair cells. If you cut them in the other dimension and look at them in the longitudinal plane, inner and outer hair cells look completely different. An inner hair cell is a big fat cell. It comes up to a neck and bulges out a little bit where the hair bundle is, way up at the top.
At the bottom of the inner hair cell, there's lots of afferent nerve endings. Maybe as many as 20 per hair cell. There's a few efferent nerve endings, but they're usually not on the hair cell itself. They're on these afferent terminals. The efferents come and get on the afferent terminals.
The outer hair cells, by contrast, are completely different. They're long, test tube-like shaped cells. Down at the bottom, they also have nerve terminals. Most of the nerve terminals in this case are efferent nerve terminals. How do we know that they're efferent afferent?
Well, if you look at them in the electron microscope-- I'll just draw a quick diagram. Here's the hair cell. Here's a nerve terminal coming up. And here is a whole bunch of synaptic vesicles in the hair cell ready to be released when the hair cell is stimulated with sound. Obviously, the transmission is going that way.
Here's a nerve terminal. Here's a whole bunch of synaptic vesicles. None over here, they're all on the nerve terminal. When the message comes down from the brain, a whole bunch of these vesicles are released onto the hair cell. OK, so by just looking at the nerve terminals in the electron microscope, you can get an idea of which direction the transmission is going. And so label them as either afferent or efferent. On the outer hair cells, there are many, many efferent nerve terminals on them.
In fact, in the 1970s, it became clear that almost all afferent nerve fibers, the ones that were sending messages to the brain, were associated with the inner hair cells, and only about 5% of them were associated with the outer hair cells. This is was a big mystery for a while, and people didn't believe it at first. It said, well, all the information going into the brain, or 95% percent of it, is coming from the inner hair cell. Huh, that's kind of funny.
There's actually more outer hair cells than inner hair cells. So what's going on? Somebody screwed up the counts, right? So it was done over and over again, and the counts came back correctly.
So how do we interpret that now? Well, about the same time in the early 1980s, a very interesting property of outer hair cells was noticed. It was noticed that outer hair cells are actually motile. They can move. And this was first discovered by Joe Santos Saatchi and others in the early 1980s.
And this discovery was made when they put a hair cell in a fluid of high potassium, lots of potassium here. There are some potassium channels in the sides of the cell. Potassium went in. When you have positive ions coming into a cell, the cell depolarizes. It might have started out at minus 80 millivolts. In the high potassium solution, it may have gone to minus 50 millivolts or maybe even 0 millivolts.
Bill Brownell and Joe Santos, when they saw this happen, they saw the cell shrink. It was, let's say, five micrometers in length before. They put it in the potassium solution, it became four. Take it out of the high potassium solution, put it in a regular solution, it lengthened again.
OK, here is a graph of those data. In this case it's a more elegant experiment where they actually measured the potential inside the cell by putting an electrode into it. So you can run this out to your amplifier and measure the electrical potential in terms of the millivolts of the inside of the cell. And by putting current down or coming out of the cell, you can move the inside of this potential whatever you want, whichever way you want.
And in this case, this x-axis in millivolts is the potential inside the hair cell. The ordinary potential is about minus 80 millivolts, about right here. Minus 180 is a huge hyperpolarization of the cell. And plus 0 up to plus 40 millivolts is a depolarization of a cell. Does everybody understand what we're doing here? We're changing the intracellular potential of the cell in terms of its millivolts.
And then we're looking at the change in length. As you depolarize the cell, the cell goes from 0 to negative values. That's a shortening in terms of micrometers of the length of the outer hair cell. This is the basal end where the hair cells are, this is the apical end where the stereocilia would be.
OK, so these hair cells can actually move. You can do this experiment with a muscle fiber and get the same result. It's a very different process, but when you depolarize a muscle cell, it contracts by actin and myosin means. This was very surprising though to see this in a sensory cell. Sensory cells aren't supposed to contract, right? They figured out that they are. They can contract.
Now, that means this outer hair cell-- and I should say outer hair cell, these are outer hair cells because when you do the same experiment with an inner hair cell or any other cell in the body, you don't get a contraction. So it's peculiar to outer hair cells. Make sure you know that. Outer hair cells are the ones that are motile.
This process became known as electromotility. OK, so when the inside voltage is changed, the length of the outer hair cells has changed. So let me give you a demonstration of this. I have a nice demonstration from Joe Santos Saatchi, who is now at Yale School of Medicine. And he made this demonstration.
And it's kind of a clever demonstration because you can see the hair cell, and it will be moving because Joe has patched onto the hair cell with his electrode. And the electrode is-- usually, in these kinds of experiments-- is put on a micromanipulator and it's bolted to the table. And because that patch pipette has impaled the cell, that part of the cell is just pegged so it's not going to move at all.
That's the very bottom of the cell. And you'll see a little bit of a ghost-like image of that. The rest of the cell is the long part of the hair cell, and then the stereocilia, or hair bundle, right up at the top. And that part is free to move. Now, what Joe has done is he's depolarized and hyperpolarized the cell using his amplifier.
But he has made that signal sync'd to a musical signal, OK? So you'll hear music on the soundtrack and you'll see the hair cell moving. The music is not directly stimulating the hair cell by moving its stereocilia as normally it would be, as when we listen to it. Instead, that music is just in sync with the electrical signal manipulating the inside of the hair cell. So I'll play that now. Once again.
OK, so here's the hair cell. This is the shadow of the electrode holding this stiff. This is the main part of the cell. These are the stereocilia right up there.
OK. So apparently Joe, lectures to medical students, says there's a reflex that goes directly from your hair cells to your dancing feet. And apparently, the medical students believe him. Anyway, that is clearly a demonstration that this electromotility is much faster than, for example, the contraction of muscle cells. Muscle cell contraction, put it in a dish, or even hair cell in a dish-- it can be very slow.
This electromotility is happening at audio frequencies, right? Some of those sounds were thousands of times per second. So in the early debate of electromotility, there were some questions of how fast this is and whether it really manipulates movement of the hair cells at audio frequency. And I think that kind of demonstration clearly shows that it does.
So what good is this? How does this help us in the sense of hearing? And what good would it be to have a motile sensory cell? Well, the idea is that these hair cells-- here again are the inner hair cells and the three rows of outer hair cells. Sound comes into the ear. It moves these membranes. It moves the hair bundles.
Motion of the hair bundles opens up these transducer channels which allow ions to come in and depolarize the outer hair cells, let's say. When the outer hair cells are depolarized, they shorten. OK, when the sound phase reverses, the hair bundle moves the other way, the channels close. The hair cells go back to their normal length. And this goes back and forth, and in the outer hair cells are getting longer and shorter.
Somehow, that motion, that mechanical energy, adds to the vibration that was initiated by the sound in a sort of an amplification mechanism. So you then have more vibration. You then have more bending of the stereocilia. You have more depolarizing of the hair cells. You have even more electromotility and sort of a positive feedback loop here.
The outer hair cells then are sometimes called the cochlear amplifier. They amplify the vibration patterns set up by sound in the cochlea. So the outer hair cells are sometimes nicknamed the cochlear amplifier.
So what good is a cochlear amplifier? Well, you have this ordinary receptor cell over here. It doesn't change its length at all, but it has all the auditory nerve fibers linked to it. Now instead of its stereocilia moving just a little bit, it has an amplifier sitting right next to it and amplifies to all these membranes. And the inner hair cell stereocilia are really now waving and back and forth.
They then send their messages to the auditory nerve fibers, which send their axons and messages into the brain. Your brain says, I hear sound. Even when it's a very soft sound, like a pin dropping-- which, without the cochlear amplifier, would be inaudible-- that pin dropping, that very small mechanical motion is amplified. And the inner hair cells then say, oh, yes I do hear the sound.
So the function then of these receptor cells is very different than what you have in vision, where you had rods and cones, right? Rods and cones mediate different types of vision. In the cochlea, the hair cells work together. The outer hair cells ore the cochlear amplifier making this thing amplified more, and more sensitive. The inner hair cells then are the major receptor cells that are sending their messages to the brain, OK? So it's really a different kind of two receptor sense, if you will, compared to vision.
And that's what this diagram is supposed to be. This is very fanciful diagram. And there's a lot of hand waving here associated with how the cochlear amplifier really works. This is an unraveled cochlea from the base to the apex, and this is how much displacement you have-- von Bekesy's traveling wave envelope, if you will.
This dashed line is what would happen if you just put sound in and there was no amplifier. And this enhanced solid line is when you have the outer hair cells working their cochlear amplifier magic. And apparently, the active region where the outer hair cells are most active is just basal to the peak of this traveling wave.
And how do we know that? How do we know that the outer hair cell cochlear amplifier is very important? Well, there are actually situations when you can lose your outer hair cells and you have pretty intact inner hair cell population.
So in an animal treated with the aminoglycocide kanamycin, it turns out that the outer hair cells are a little bit more sensitive to the kanamycin then the inner hair cells are. So if you treat with just the right dose, you can find a place of the cochlea where there are intact inner hair cells and where the outer hair cells have all been lesioned.
Basal to that, for example, all the hair cells are gone. And apical to that, none of the hair cells are gone. So in a certain region of the cochlea with just the right dose of kanamycin. And in those areas, where you just have inner hair cells without the outer hair cells, you have a huge hearing loss. You're not deaf. The inner hair cells are still there and they're sending their messages to the brain by their auditory nerve fibers. But there's a big hearing loss because you have lost the function of the cohclear amplifier.
And those experiments were done in the 1970s and '80s. And they were criticized by saying, well, anytime you do some lesion treatment, you say you have normal inner hair cells and outers. Well, you don't really know the inner hair cells are normal. Maybe the drug affected them. They're still there, but they're screwed up.
So recently, a much more elegant way of doing that same sort of experiment has come up. And this is the paper, the research paper, that is assigned reading for today's lecture. It turns out you can knock out the cochlear amplifier by knocking out a particular protein in the outer hair cells and have the outer hair cells still there.
And this work started out with the discovery of a protein that's in the membrane of the outer hair cells. So if you look at the outer hair cells, there's a lot of protein in the membrane. And the protein is found pretty much nowhere else in the body and was given the name prestin.
Now, you amateur musicians out there, when you play a piece-- right?-- at the beginning of the piece, at least for classical music, the composer gives you an Italian word that says how fast you should play it, right? And if it says largo, you're supposed to play it really slowly, Right or adagio, slow, right? What's the marking or Italian word for "fast"?
AUDIENCE: Presto.
PROFESSOR: Presto, right. And this protein was named prestin because, at least at the time it was discovered, they had the idea that, oh, OK. Maybe it's the cochlear amplifier protein and it makes these outer hair cells shorten and contract really quickly, very fast. So we'll call it prestin.
It turned out that that was true, and here's some of the evidence in support of that. You can knock out the gene for prestin. So a knock-out is an animal in which a particular gene is either removed or made so it doesn't make the protein. Part of it's deleted, and so the protein is not made. You can make a knock-out mouse where the prestin is knocked out. And that's what was done in this paper.
OK, and in that knock-out mouse, you can look-- and they looked at a whole bunch of things. That looked at the electromotility the outer hair cells. So they took outer hair cells and put them in a dish and looked at the kind of movements we saw in that video. And in this trace, this is minus minus, which is the geneticist lingo for a knock-out. Both genes for prestin are gone.
This trace is the plus plus, the wild type, or normal. And there's big changes in outer hair cells when you depolarize and hyperpolarize them. And these big changes are on the order of half a micrometer. So this is a length axis.
In the knock-out, the outer hair cells don't change length at all when they're depolarized and hyperpolarized. In the heterozygote, which is the case when you have one gene intact for prestin and the other gene is knocked out, it's an intermediate result. OK, so the outer hair cell motility is knocked out by knocking out this one protein. So that's pretty good evidence that it's involved in the cochlear amplifier.
What else was measured? Well, they wanted to measure hearing sensitivity. And so in humans, what you might do for that is put a subject in a soundproof chamber and say, raise your hand when you hear the sound. But you could do that in mice, but it takes a long time to train mice or other experimental animals to do those behavioral tests.
So they did an electro physiological test. They measured what's called the auditory brain stem response, so the ABR. This stands for auditory brain stem response. OK. And that top right graph gives you the ABR threshold in dB. So something that has a really low threshold is a really good hearing animal. This is the wild type and the heterozygote.
And something that has a very high threshold means you really had to crank up the sound to get any kind of response at all as you see in the open symbols for the knock-out. So how do they measure that ABR? You could do it in animals or humans as a clinical test. Put electrodes on the surface of the skin. You turn on a click or a tone burst. In this case, they used tone bursts of different frequencies.
And you can imagine that the auditory brain stem is way down in the head, and you're measuring on the surface. So you've got a click, click, click. And you measure thousands of responses. So there's a lot of noise. There's noise in the room. There's noise from other neurons in the brain. But eventually, after thousands of averages, that little tiny signal comes out of the noise, and you get a response if the brain stem is responding-- that is, if the hair cells are responding, the nerve fibers send messages into the brain stem, and the brain stem finally responds. It's a very good test of auditory sensitivity.
And what does it show? It shows that without prestin in the knock-out animal, you have a huge hearing loss. How big is the hearing loss? Well, looks like it's about 40 to 60 dB. So when you have prestin knocked out, you have a hearing loss of 40 to 60 dB. How much amplification does the cochlear amplifier give you? 40 to 60 dB.
You're not completely deaf without it, but you have a severe hearing loss. Most of you would not be able to understand what I'm saying with a 60 dB hearing loss, unless you were sitting right up here in the front. What else do I want to say about this paper? Not too much. There are some problems with it. Any paper has problems.
They found that, for some reason, all the hair cells were lost for the high frequency basal part of the cochlea. It's just a problem in some strains of mice that they lose hair cells in a certain part of the cochlea. So within these gray bars, you can't conclude anything.
Now, they looked at the hair cells. They just took them out and looked at them in the microscope. And they said, wow, in the knock-out, the hair cells are actually smaller. Well, you cut out all this protein from the membrane in the knock-out, right? So if there's a lot less membrane there, they shrink. The outer hair cell membrane is packed with prestin.
So you could argue, oh, all the hair cells are shorter, and so they're not working the same way. Every paper has its problems. but that's what these graphs mean. The hair cells at rest are actually shorter in the knock-out. So just some caveats. I think the main message is prestin is essential for the cochlear amplifier. And without it, you have a big hearing loss, 40 to 60 dB.
Now, one of the other things they measured in here is something called the distortion product otoacoustic emission. And let me just, as the last thing in today's lecture, tell you about what an otoacoustic emission is. These were discovered about the same time as outer hair cell electromotility by David Kemp, who's at University College London.
And he was doing some kind of hearing tests in people, and he developed a very, very sensitive microphone that had a very low electrical noise. He stuck that microphone in an ear canal. And what did he find? The microphone actually picked up sound in the ear canal. Oh my gosh, this is crazy. There's not supposed to be sound coming out of the ear, you're supposed to be putting sound into the ear, right?
So he named it otoacoustic emission. OK, "oto" means ear. "Acoustic" means sound, and "emissions" means coming out, sound coming out of the ear. This was an amazing discovery, and it fits very nicely with the idea that there's something in the ear that's actually moving, that being the outer hair cells. The outer hair cells are moving either spontaneously-- and there are some otoacoustic emissions that are spontaneous. About half of us have spontaneous otoacoustic emissions in our ears.
Now, before you get too excited and go home and listen to them, they are very, very low levels of sound. Most of them are below the audio metric hearing curve for human hearing. So you really, in most cases, are not aware of your otoacoustic emissions. This is very different from the sensation that some of us have of ringing in the ears, tinnitus.
Does anybody have tinnitus? I have tinnitus, especially my left ear. If I close my left ear, often I can hear kind of a noise. Put my head on the pillow at night, I can hear a little noise in there. So that's a sensation that I have even though there's no sound going in my ear, and it's not an otoacoustic emission. You could put a microphone in that ear, and there is no sound there. Something in my brain is telling me that I am hearing a sound.
Some people are very disturbed by tinnitus. There's no good treatment for it. Historically, the famous treatment was by an ear surgeon who said, OK, I'll cure your tinnitus. And he took out the person's ear, the cochlea was taken out. Tinnitus didn't change one bit. Maybe it's like phantom limb pain, something to do with your central nervous system.
Tinnitus and otoacoustic emissions are completely different. Otoacoustic emissions are associated with normal hearing, normal outer hair cell function. They're sometimes used as a clinical test for hearing in patients who can't raise their arm. For example, most states, like Massachusetts, you have to, by law, test newborns for good hearing. An otoacoustic emission test is one.
So how does that work if only 50% of the people have them? Well, there are other types of otoacoustic emissions that are evoked-- that is, you put sound in, and you listen for the sound coming back out. Some of these are transiently evoked. You put a click in, and a few milliseconds later you get a sound coming back out. And that's the usual clinical test. And 100% of normal hearing humans have these so-called otoacoustic emissions.
Now, what would be an indication if you had a patient with no otoacoustic emissions? Well, it is a good test of whether your middle ear and inner ear is working as far along the pathway as the outer hair cells. Beyond that, it doesn't test. It just tests up to the electromotile part of the hearing organ. So it doesn't test the inner hair cells. It doesn't test the auditory nerve fibers.
But much of hearing problem arises in the outer hair cells, so it's a pretty good first step. It's a very easy test to do. And I think when we have the lab tour over at the Mass Eye and Ear Infirmary at the end of the semester, we'll be seeing some otoacoustic emissions recorded from a human. There's a project going on there now. So we'll have a demo of that.
OK, so if there aren't any questions, we'll meet up again on Monday.
Free Downloads
Video
- iTunes U (MP4 - 188MB)
- Internet Archive (MP4 - 188MB)
Subtitle
- English - US (SRT)