This section contains documents that are inaccessible to screen reader software. A "#" symbol is used to denote such documents.
Flash and JavaScript are required for this feature.
Download the video from iTunes U or the Internet Archive.
Topics covered: Nuclear Chemistry and the Cardiolite® Story
Instructors/speakers: Prof. Christopher Cummins
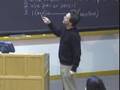
Lecture 36: Nuclear Chemist...
The following content is provided by MIT OpenCourseWare under a Creative Commons license.
Additional information about our license and MIT OpenCourseWare in general is available at ocw.mit.edu.
I didn't quite get a chance to tell you a few things about photosystem II last time, so I am going to go ahead and finish up with that now before going onto the Cardiolite story.
Photosystem II shares quite a lot in common with photosystem I, but there are a few major differences.
Photosystem II is the first link in the chain of photosynthesis. It is really where all photosynthesis starts out on earth.
And, as such, it is an extraordinarily important enzyme, this photosystem II.
But it is such a large and complicated enzyme.
And it is also a membrane enzyme.
As I mentioned last time, these are difficult to isolate and purify and crystallize so that you can study their structures. And it was only in the last couple of years that photosystem II was actually crystallographically characterized, so there is really a lot of very recent science that is relevant to today's presentation on photosystem II.
As you go through and read about it, you are going to learn about the molecules that harvest the light, and you are going to learn about where the electron goes and where the hole goes when light is absorbed by this amazing system.
Here is a close up of part of the system called the reaction center, for obvious reasons. This where the reactions take place that are so integral to photosynthesis.
Namely we have, up here at the top, a manganese-containing cluster, the structure of which is still a little bit ill-defined. As you can imagine, with a macromolecule of this size, it is difficult to say exactly where just each and every atom is in the structure.
There are still some details of the structure, of the manganese cluster, here, that are not known.
But, nonetheless, this is where two water molecules must be brought in and coordinated and ultimately where water, which is not a good reducing agent, serves as a source of electrons in this system.
And what happens is this special pair of chlorophyll molecules at the heart of the reaction center absorbs a photon and goes into an excited state. And the electron is able to travel down through a series of pigment molecules, and it gets down onto this plastoquinone A.
And it is able to jump over onto the plastoquinone B.
And plastoquinone B then can dissociate from the reaction center and carry that electron onto the next link in the chain in photosynthesis. And, at the same time, the hole is stuck back over here and ends up accepting an electron by oxidation of a reduced tyrosine moiety here.
This tyrosine has a phenoxy residue.
It is connected to the amino acid polypeptide backbone of this protein and it is positioned right here between the manganese cluster and the special pair, so that once the electron is shuttled away down this redox cascade pathway, it is juxtaposed with this reduced tyrosine, which is getting its electrons from the water that is coordinated to the manganese complex. And somehow those two water molecules, after giving up their four electrons, become O two. And O two gas bubbles out, and that is the source of the oxygen that we breathe on this planet. So this is, needless to say, a very important enzyme to understand.
And I won't have time to go through an exploration of the structure today, but I will lead you into it by showing you this picture. And in this picture, the sort of gray background that is mapped out here on the outside represents this polypeptide chain, all the amino acids that are linked together to form the protein container of photosystem II.
And you can see that it looks like a dimeric structure where you have one reaction center over here, here is a special pair located over there. And then all embedded throughout this protein you find many, many cofactors which are like porphyrins, but contain magnesium.
And there are these chlorophyll units.
And then also, shown in sort of an orange color here, you see these long straight molecules.
These are polyenes. These are carotenoid molecules, organic molecules that have strings of double bonds adjacent to one other so that they can conduct electricity and conduct energy. And this is a really key feature of this -- -- because the point that is being made here is that the photosynthesis does not wait until a photon actually comes in and impinges right there on the special pair. A photon can come in anywhere on this big molecule or on these light harvesting proteins shown at top and at bottom that come up and dock next to photosystem II. And these light harvesting proteins are also just chalk full of light absorbing chromophores, chlorophylls, and carotenoid molecules. And, if a photon comes in and excites one of these molecules over here, the proton has a mechanism for transmitting the energy over to the special pair, so that water can be oxidized to O two.
It is really quite a tremendous assembly of different kinds of molecules to perform function. And if you go on and take 5.03, for example, and then 5.04, you will learn about the rules that govern electron transfer between molecules and also in proteins.
Let's see. Finally, on this subject, I will show you, here, a view of this reaction center. And this just a close-up to show you that these water molecules indeed must get in here. There is also a calcium ion present at this active site, where the water molecules are being oxidized and converted into dioxygen.
And here is that tyrosine residue shown in more detail.
That is very important for the hopping of the electron from water to the tyrosine. And then over here, after the hole has been generated through excitation of the special pair of chlorophyll molecules.
And so that brings me to the end of my discussion of six-metaloenzyme systems that had quite a bit in common in terms of the ligands that are used to bind metals.
We talked about heme last time and its employment of the porphyrin ligand in this regard. But then, you should also remember that sometimes you see clusters packed into protein molecules as cofactors. We saw last time some Fe four-S four clusters, and here, a manganese cluster.
And so inorganic and organic molecules are all working together in a beautiful synergy to enable photosynthesis.
And, having said that, I would like to now go onto my final topic, which has to do with the role that metals can play in medicine. Last time was metals in biology. Now, we will talk about metals in medicine. And there are different ways that metals can be used in medicine.
For example, there are molecules like cisplatin that are used in therapeutic methods for treating cancer. Alternatively, metals can be used in a diagnostic manner.
And that is what I will be talking about here today.
And so what I did for today was I went directly to a couple of the Ph.D. theses that are in the MIT digital archive by two students who worked in the Chemistry Department here and earned their Ph.D.
theses doing the chemistry of technetium.
Now, I like technetium for a lot of reasons.
One, it is a neighbor element to molybdenum in the periodic table. Number two, it is named after MIT, so that is wonderful. Number three, technetium is unique as an example, right in the center of the periodic table, as a manmade element.
And it has been known for quite some time, and I am going to tell you a little bit how technetium chemistry has evolved in the MIT Chemistry Department. And so this first thesis is a 1983 thesis of a fellow by the name of Mike Abrams, a very smart graduate student who was given a very difficult research problem indeed for his Ph.D.
thesis. And that had to do with preparing compounds directly from the pertechnetate ion.
And pertechnetate is the ion TcO four minus.
And, if you look through this, you will see why Mike Abrams needed to prepare compounds directly from the pertechnetate ion. And it has to do with the idea that there is an isotope of technetium.
An isotope known as technetium-99M, M for metastable, that has a six hour half-life.
And, in that six hour time window that is the half-life of technetium-99M, it is dropping from a nuclear excited state into a nuclear ground state.
And I will talk more about that in a moment.
But as the nuclear excited state relaxes to the ground state, this nucleus emits a single gamma photon at about kiloelectron volts. And so that single gamma photon, high energy photon, that comes out when this nucleus decays from 99M to regular technetium-99, which has a much longer half-life on the order of 10^5 years, is what is useful in a branch of medicine known as nuclear medicine. So radiologists will be interested in this. And, as you will see, cardiologists will be interested in this as well.
And so, because of this, gamma camera images can be obtained at high-resolution to image organs that have taken up this radioisotope. Let's point out also, here, that the chemical form of technetium obtained from the generator that creates it is the pertechnetate ion, so all radiopharmaceuticals have to be prepared from TcO four minus because that is what you get.
Molybdenum-99 is a product of nuclear fission.
And so, when people are using nuclear reactors to make energy, they are accumulating a lot of molybdenum-99.
And molybdenum-99 is an unstable isotope of molybdenum, and so it is right to the left of technetium on the periodic table. And molybdenum-99 itself undergoes a beta decay, in which a neutron turns into a proton and electron. And that beta decay converts molybdenum-99 into this metastable isotope of technetium. So what happens is that every morning at the hospital, the molybdenum-99 has been coated as MoO four onto an alumina column and is undergoing its own beta decay. And when that is done, you now have pertechnetate on the column.
And you can elute that in very dilute solution, in isotonic saline solution. You then have a very small amount of time to do chemistry with it before you put it in the body. Therefore, Mike Abrams, and I will tell you about the professors involved in this in a little bit, was given the problem of finding a way to take an extremely dilute solution on the order of 10^-9 molar solution in saline and do a quantitative reaction from pertechnetate to make something that would go and localize in an organ that you want to image to find out about the patient's health. That is a tall order, but that didn't stop them from looking into this.
In fact, I included this page in part, because Mike Abrams has some nice statements, here.
He says the reason we carried out this research was to gain a better understanding of what kinds of coordination complexes can be prepared directly from TcO four minus with the hope that such information might lead to the development of new radiopharmaceutical agents and/or to a better understanding of the agents already in clinical use.
This thesis was written in Well, let me not get ahead of myself here.
And let me just show you here one of the first technetium complexes that Mike Abrams was able to prepare.
And this is a technetium hexacis thyourea species.
You will see that he has this pertechnetate and was able to convert it into molecules that are octahedral at technetium.
And, in fact, most of the applications of technetium in nuclear medicine do involve octahedral six-coordinate technetium, -- -- harkening back to what we can understand based on the efforts and achievements of Alfred Werner.
Here is the octahedron, pretty soon, going to go into a patient's body.
Here he has chosen to use sulfur donor ligands, these thiourea ligands in making this.
And he also made molecules in which phosphines and phosphites were able to bind to technetium. And later, as you will see, he also did work with isocyanides.
This is 90 degrees rotated. Basically, the reason I am showing you this is that this is a nuclear magnetic resonance spectrum of a technetium-99 compound.
Let me just explain that initially, when doing these studies, Mike Abrams was working mostly with technetium-99 that has the real long half-life so you can actually make molecules on a scale sufficient for isolation and purification.
But then those methods would be translated to working with the 99M isotope. And the difference between 99 and 99M, has to do with the spin of the nucleus.
We have talked about electron spin.
Well, different nuclei have themselves the property of spin.
And technetium-99 has a spin one-half nuclear spin.
And then when it decays from 99M to 99, the 99 isotope has a spin nine-halves, actually.
And that is the lower energy state that gives rise to this gamma photon emission. And so, in the nuclear magnetic resonance spectrum, observing the phosphorus 31 nuclei that are connected to this hexakisphosphate of technetium 1, you see that there end up being ten lines. And that is because of the spin nine-halves ground state of the technetium-99 nucleus.
You will see that back in the `80s, we were looking at technetium-coupled phosphorus NMR spectra, as well as, you saw X-ray crystallography a moment ago.
This was real nice work. And let's see what I have here.
After Mike had successfully synthesized some of these complexes they started wondering, well, can we image organs with these. The idea was that radiopharmaceuticals that had been in use prior to this time did not really have ligands on the metal.
It was sort of the case that they would take the metal in whatever form they got it off a generator and just inject it directly into the patient. And, in that case, you don't have an ability to tune the properties of the metal to have it localize specifically at organs that you might want to image. And so they thought we are coordination chemists. Let's put ligands on there, and you can use different ligands.
And then, the molecule will go to different parts of the body specifically. And, when we find the right ligand, we will be able to do something useful, like image the heart. And so here, they had an anesthetized dog that they had injected with a solution of one of these technetium complexes.
This was a hexakis isobutyl isocyanide technetium-99M.
And they were able to show that this stuff forms quantitatively, even at the tracer levels that they are using to 10^-9 molar.
Very dilute solutions. And so you don't actually get much radioactivity put into you, but because of the high energy nature of the gamma photons that come out, you can easily image them with a gamma camera and you can reconstruct the position from which that radiation is originating.
That is what allows you to image an organ.
And so here is a little schematic of the ribcage of a dog. And you can see that he is telling us where the liver is. And then you are supposed to be able to see kind of a doughnut, which is the myocardium of the heart here. And you see it lighting up real nice, right here. So right away, with one of the first complexes that they made, they found that this stuff goes shortly after injection and localizes in the heart and gives you a pretty nice image of the heart. And there are different viewpoints that you can take when you image the heart using methods like this, but this was already pretty exciting that one of the first systems they made, these t-butyl groups on it, were very nice imaging agents for a dog. And then the basis for the nuclear cardiology application of this chemistry is that if a person has had a heart attack or is at-risk to suffer a heart attack, has some heart disease, then what happens is you look at the heart and it doesn't become very well-perfused with blood all the way around, as it should in a normal healthy heart. And so, if there is infarcted tissue, you see an image like this, where part of the doughnut is not lighting up. And that means that part of the heart is not getting suffused with blood.
And so this kind of diagnostic test is called myocardial perfusion imaging, because you are looking at how the heart is taking up blood in all of its different parts because you can look at different angles of view.
And one also tends to do this when the subject is at rest versus when the subject has been stressed.
So you will hear the term stress test associated with this type of image. And so this is really the first complex that Mike Abrams made that they were actually testing in animals. Here was an infarcted dog heart. You see that there is blood not getting to this part of the heart, up here.
And this image was to be compared with an image made using a different imaging agent which was the state of the art at the time, here, with just the first one.
And there were so many choices you could make of what ligand to put on this metal. And the first one already works better than thallium-201 that is inserted just as an aqueous solution. So thallium-201 is also a popular isotope for applications in nuclear medicine, but with technetium, now, you have the ability to make coordination compounds where the ligands are very tightly bonded to the metal center.
And then you can attach other residues to this system by virtue of how you build the ligands.
That was really pretty amazing. And then a few years later, also in the research group of Alan Davison, Professor of Chemistry at MIT, comes along one James Frederick Carnegie. And what he decided to do for his thesis work was to go ahead and actually make molecules like the ones that Mike Abrams made, but to look at many different varieties with different peripheral substituents because there are certain organic functional groups that will be metabolized and chopped up by enzymes in the body.
These include ester groups, for example.
You can also attach to these isocyanide ligands things that look like little proteins. And you can see, then, how those react to being injected.
And so his goal here was really to make something practical that would be useful as a radiopharmaceutical for imaging organs. And his discussion begins by just talking about the different groups that he was going to append to the surface of his coordination complex.
And then, here is a little synthesis slide from his thesis.
Mike Abram's thesis, the first one I showed you, was actually typed up by hand on a typewriter.
But Jim Carnegie's thesis was prepared, he tells me, I was talking to him last night about this, using a very primitive word processor. One of the first-generation word processors. Anyway, that is a little bit beside the point. You just don't see as many nice graphics in these theses as you do nowadays, but you do see a lot of beautiful chemistry. Here is an isocyanide ligand.
Look. We have talked a lot about carbon monoxide in this class as a ligand in its behavior to transition metals. And so if you just change the oxygen of carbon monoxide into a nitrogen, then you can have a substituent stuck to it. Here is an example of an isocyanide ligand where the metal is going to attach to the carbon. And then, Carnegie decided to put other groups out here. Here, he has a carboethoxy residue. And the idea was that enzymes called esterases in the body can clip off and metabolize the carboethoxy residue. And what that will do is it will affect the way that the technetium is bio-distributed in the body. It will affect the kinetics of how long the technetium takes to accumulate in an organ and how long it takes to then run out of that organ.
And also, he looked at examples that have organic amide functional groups, all connected to the isocyanide. His thesis work was all about looking at different isocyanides, six of them on technetium in the +1 oxidation state.
It is d^6 low-spin octahedral diamagnetic systems.
It talks here about the radioactivity.
It talks a little bit about the technetium generator issue.
It mentions that he is doing a lot of this work in collaboration over at Harvard Medical School.
They had a lab over there where they were cleared to use 99M.
The 99 work was done here, at MIT.
And this was because of the collaboration that I will tell you about shortly. Here is one that I won't bother to turn over. But this actually a pretty important slide. You will get this pdf file from our website, but you will see that he is taking pertechnetate and saline. The key feature, here, is that this synthesis is quantitative at these very low concentrations that are what used for nuclear medicine.
Now, I showed you that Mike Abrams imaged part of a dog.
And as they progressed toward doing human studies, they also imaged the rabbit. And in these early tests, you know, you have these chemistry Ph.D.
students who don't really know which organ is which in a bunny.
They had to get a diagram like this, out so they would have a map, so that when they started shooting these molecules into the bunny they would know which organ they were seeing light up by the gamma camera. And this does not hurt the bunny. The bunny is anesthetized and does not know, so this is a perfectly nice thing to do. And this one, I think the bunny is oriented this way, here is the liver.
And resting atop the liver on the diaphragm is the heart of the bunny right here. He has a lot of different images. These, in the digital thesis archive, are a little bit fuzzy. If you want to see better images, you might want to refer directly to the thesis.
But you will see that what he is doing here is he is looking as a function of time after injection, just the intensity of the gamma counts from the liver, from the kidney.
Notice the heart starts out real high, and then it clears as the blood moves on into the kidney and the liver.
And so you can actually take these images as a function of time and see where the technetium is going in this living bunny. And I thought I would mention that. But then, I thought I would also show you this picture. I have seen this in the real hard copy thesis, and it looks much better in terms of organs lighting up and so forth.
It is very fuzzy in this image from Carnegie's Ph.D.
thesis. This, in fact, is Professor Alan Davison, who was himself the first test subject for his technetium radiopharmaceutical.
He just couldn't wait to have this stuff shot into him, so he could see what his organs looked like when imaged with a gamma camera. That is Professor Davison, first human test subject on his own chemistry.
That was pretty remarkable. Let me tell you just a little bit more about Alan Davison. You can go and read about him at the MIT website, but this is Professor Davison.
He is a colleague of mine in inorganic chemistry, here at MIT. He became an emeritus faculty member this past summer, but his research career spanned 40 years here at MIT. He came in 1964.
And he did research in a lot of different areas of inorganic chemistry, and did not get into technetium until 1980 because he struck up a collaboration with the fellow at Harvard Medical School, who I will show you next.
He is a Welshman. He got his Ph.D.
with the Nobel Laureate Geoffrey Wilkinson at Imperial College, London, after Jeff Wilkinson was denied tenure by Harvard, after which he got his Nobel Prize. They did not make a very good choice, there. But, in any event, Professor Davison learned and became one of the top inorganic and organometallic chemists of his generation.
And, in fact, he is now a Fellow of the Royal Society, which is quite a special distinction.
I went over there for the ceremony in London when he was being inducted into the Royal Society.
And they pull out the book that is signed by all the new inductees into the Royal Society.
And one of the early signatures in the book is that of Isaac Newton. So he signed the same book as Isaac Newton. You have to make sure your hand does not tremble and blot the page.
It would be a big problem. They actually go ahead and teach them how to sign the book and practice in advance because they use a quill pen dipped into ink, just the way Isaac Newton did it. And this coming spring, Professor Davison is going to Atlanta, as many of us chemists are, for the American Chemical Society meeting there.
But he is going for a special reason.
He is winning the American Chemical Society Award for Creative Invention, which is a very prestigious award. And I believe he is the first from our department ever to win the Award for Creative Invention. And that can go to a person in any area of chemistry, in fact.
That is a really nice thing. And Professor Davison was a great mentor of both undergraduate and graduate students here at MIT over the years.
And next let me show you that it takes not one Welshman, but two, to make a drug. And here is the other one, Alan Jones, a very good personal friend of Alan Davison.
He and Alan got together in the early `80s to see if they might not be able to bring inorganic chemistry and coordination chemistry to nuclear medicine in order to develop a diagnostic imaging agent. They got together, and their research was extremely successful.
And they have done research in other areas, too, together over the years. And their students go back and forth between the labs here and Building 6 at MIT and over at Harvard Medical School to do the different kinds of studies that they do. It turns out that his chemistry, making these six-coordinate complexes of technetium-1, has led to the development of Cardiolite. This now is called Sestamibi.
That is an abbreviation for this compound that has six of these isocyanide ligands on technetium.
When you see Sestamibi, you will know what molecule that is. It is the one that actually became commercial and was approved by the FDA in after extensive critical trials. I will show you the structure in a moment, but it is a variation on the t-butyl, where one of the methyl groups is replaced with a methoxy group. It is a methyl-ether species.
And Cardiolite was originally manufactured and brought into being by DuPont Merck. And they sold it later to Bristol-Meyers Squibb. And so you will see that Bristol-Meyers Squibb has a section devoted to medical imaging. And this drug that was the brainchild of Davison and Jones is currently the most widely used diagnostic imaging agent for looking at myocardial perfusion. It is used in heart imaging tests all around the world, every day, so it is a very important molecule contributing to the health and welfare of people all over the world. And what you will see is there are here descriptions of the stress tests that people take.
Let's see. Cardiolite imaging guide.
You have education programs. Let's just check out the imaging guide for a moment. These companies have pretty good graphics on their websites. And remember the doughnut I was showing you, there it is right here.
There are different ways to look at the heart using Cardiolite to see if the blood is perfusing the heart properly.
That might be the short axis. You can view the scan images.
This is how a normal healthy heart will differ when it has been stressed after exercise or at rest.
That is why they call these stress tests.
You are seeing how the blood is distributed in the heart using the property of the radionuclide technetium-99M.
There is one. That is a different one.
I am not going to spend too much time on this, but I am giving you the link to this website so you can go and look at how people actually use clinically technetium Sestamibi to image the heart. I want to show you just a few other things here, one of which is that, as you may be aware, the same molecule can have different names if it is used for different purposes.
Cardiolite is one of the names given to Sestamibi, but another name given to Sestamibi, here it is, is Miraluma. And Miraluma, it turns out, is really quite useful at detecting breast cancer when breast lesions are not readily visible using mammography or ultrasound.
If you go to this website and look at the information for healthcare professionals, as you all are sitting here, you will be able to see breast tumors that are detected very nicely using Sestamibi that were missed using ultrasound or mammography. And you might like this.
If you try to go into that information, you're first confronted with a test. And you have to pass the test and know that the risk of breast cancer increases with age.
If you get that right, then you can go on and actually look at the images that are made using this drug.
Now, I also give you a link here to the Cardiolite story that is a nice article written in the MIT Undergraduate Research Journal about Professors Davison and Jones, their students, and the creation of this clinical tool. You can go there and read that.
And it has some more information.
And then, finally, I do give you a link to Sestamibi. You will probably be appalled at the way this website draws the isocyanide complex of technetium. We know very well that that is a linear bond angle at those carbon atoms, not bent like that, so that is not a very good representation in terms of structural fidelity.
But if you want to know which isocyanide is used in vivo for imaging organs, it is this one with the methoxy group, instead of one of the methyl groups of the t-butyl group. It is very similar to the original one that Mike Abrams made first back in 1983 because they already had t-butyl isocyanide around from other things. It is a really remarkable story, a fantastic story. And having told it to you, I am now going to have to do something that I very much regret. Now you can go on and sample all the other exciting chemistry subjects at MIT in your future.
I hope to interact with many of you again in that context.
And I wish you good luck. I know that you all will do fantastic things. Having said that, I am going to have to close the book on this semester.
[APPLAUSE]
Free Downloads
Video
- iTunes U (MP4 - 81MB)
- Internet Archive (MP4 - 146MB)
Audio
- iTunes U (MP3 - 8MB)
Subtitle
- English - US (SRT)