Flash and JavaScript are required for this feature.
Download the video from iTunes U or the Internet Archive.
Description: In this lecture, Prof. Liu discusses how to calculate the entanglement entropy of a boundary system using the AdS gravity. He gives a holographic proof of the strong Sub-additivity conditions of the entanglement entropy, and discusses a simple example.
Instructor: Hong Liu
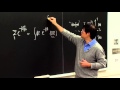
Lecture 24: Holographic Ent...
The following content is provided under a Creative Commons license. Your support will help MIT OpenCourseWare continue to offer high-quality educational resources for free. To make a donation or to view additional materials from hundreds of MIT courses, visit MIT OpenCourseWare at ocw.mit.edu.
HONG LIU: So first, do you have any questions regarding this Hawking-Page transition we talked about last time because we were running out of time, so it was a little bit hurried. Do you have any questions about that?
AUDIENCE: So what's the original thermal AdS stat? Did you put some random [INAUDIBLE] onto that metric, and it will generate some [INAUDIBLE]?
HONG LIU: Sorry? Say it again?
AUDIENCE: So what's the original thermal AdS state?
HONG LIU: The thermal AdS state-- you couple AdS to a thermal bath. And then whatever excitation will be generated by that thermal bath will be generated. So mostly it's the graviton gas. It's the gas of the massless particles inside AdS. So that essentially. The field theory procedure to go to cleaning space and to make the cleaning time periodic. And that physically should be interpreted as you just a coupled decisions to your thermal bath.
AUDIENCE: I have a question not really related to this. But I'm just wondering why do we want to consider [INAUDIBLE] on a sphere, because previously we discussed about [INAUDIBLE] 4, which is reality. What is this?
HONG LIU: So normally when we look at the theory, you want to look at the theory from as many angles as possible. So some of them may not be able to directly realize it in the experiment. But still, it's useful from a theoretical perspective because that gives you additional insights. So we see that the physics on the sphere is actually quite rich. So that actually gives you some insight into the dynamics and also into the duality itself. Yeah.
AUDIENCE: So there's no condensed matter system essentially like that.
HONG LIU: Oh, if you're talking about condensed matter applications, you may even imagine in some systems, you may be able to put it on a sphere. Certainly, you can put it on a two-dimensional sphere. And I can imagine you can put it-- you can manipulate say, and put some spins on the two-dimensional sphere. Yeah, you might be able to do that. Any other questions? Yes?
AUDIENCE: [INAUDIBLE] you said there are two kinds of [INAUDIBLE] connected on the same [INAUDIBLE].
HONG LIU: Yes.
AUDIENCE: So it's like two CFTs?
HONG LIU: No, it's a single CFT. It's just a different sector of the CFT contributes.
AUDIENCE: So it's like 0 quantum critical point [INAUDIBLE]?
HONG LIU: No, this is a first-order phase transition. So it's not the quantum critical point. So the picture is that you have a temperature. So it's some TC. So below TC, you have a phase which we call the thermal AdS phase. And in this you have a big black hole phase. So translated from the field theory side-- so this goes running to the states which energy scales into the power 0 contributes.
And here, it's dominated by the state of energy O (N square). Of course, in the thermal ensemble, every state in principle contributes. And in this phase, it's dominated by the contribution of the no energy state. And here, it's dominated by the high-energy state. And really the high-energy state also has a much higher entropy. And then they dominate your thermal ensemble. Does this answer your question?
AUDIENCE: Yeah, this looks like in any transition, you have [INAUDIBLE] carry energy and also [INAUDIBLE].
HONG LIU: Yeah, it is a little bit similar to that. There's also an entropy thing there. Yeah, it's a balance between two things. But the details is very different. The details are different. There's some qualitative similarity.
AUDIENCE: So I read on the paper [INAUDIBLE]. He said that the Hawking-Page transition on the field theory side is due to some kind of deconfinement and confinement of QCD.
HONG LIU: Yeah, so that's a heuristic way to say about it. So the key thing is that here, it's not really a confinement or not confined because in the [INAUDIBLE] series, there's no confinement. It's just scaled in [? Warren's ?] theory. And so in the sense he says this is confined-- so he called this a confined phase. And this is a deconfined phase-- it's based on the following. It just refers to these two behaviors.
It just refers to these two behaviors. So in QCD, which will generating confinement or deconfinement-- so in QCD, we have [INAUDIBLE] 3. But if you scale into infinity, then you will find in QCD in the confined phase, the free energy will scale as N with N power 0. But in the deconfined phase, which is scale-- obviously the N square. So that aspect of scaling is the same as a serious risk confinement.
So that aspect is similar. So he essentially refers to this aspect. But on the sphere, every state has to be a singlet. So in some sense, this notion there is no genuinely notion of confinement as we say in the QCD in the flat space case. If you read his paper, he actually described this very clearly. Yeah, he just called this a deconfinement transition the heuristic way. Yes?
AUDIENCE: I have a question about the sum by which we calculate partition function. The previous class on Monday, you wrote it as sum over energies. The degeneracy of that [INAUDIBLE] factor. And you noticed that both of these grew at exponential to something times N squared. One was positive, and one was negative. I was wondering if it's possible that sum diverges?
HONG LIU: No, that sum does not diverge. That sum is always proportional to n square. Yeah, depending on what you mean the sum diverges. So that's just from the definition of your partition function. So it's just the sum of all possible states. And then you can just reach this-- yeah, when you have a large number of states, you can just roughly write it as a continuous integral and then with the density of states.
And so the key is that what happens to the density of states-- so you're asking maybe whether this integral will diverge when it equals infinity. So when it equals infinity, you will see that say supposing E scale as N cubed. Then you will see that the density of state actually does not grow that fast. And actually, it's only for the E of O(N squared), then they scale in the same way. They scale in the same way. So that's why you have this balance. And if you are not in the scaling with [INAUDIBLE]. If you have N cubed, and then this will dominate. So that factor will be suppressed.
Any other questions? OK, very good. So now let's go to entanglement. So first, let me say a few words about entanglement entropy itself. So this is very elementary stuff, even though maybe not everybody knows. So let's consider a quantum system, just a general quantum system.
So let's separate the degrees of freedom into two parts, say A plus B. So AB together is the whole system. We just separated the degrees of freedom. So essentially by definition, the Hilbert space of the system-- then we'll have a tensor structure. So the full Hilbert space will be a tensor product over the Hilbert space for the A part and tensored with the Hilbert space in the B part. OK?
And consider, of course, in general, we have an infinite dimensional system. But in the case if you have say a [INAUDIBLE] Hilbert space-- so i f this M dimension, this is N dimension, then the total Hilbert space will be M times N dimension.
And the typical wave function will have the form-- will be some sum-- say you can write it in some basis in A and times some wave function writing some bases in B. And this actually means that the Hilbert space is a tensor product. It's just your wave form can typically have this form.
So we say that AB-- so we say that in the states psi that AB are entangled if psi cannot be written as a single product-- rather than the sum of product and if you just have a single product.
So for a simple state, it might be easy to see. But if I write the very complicated state which many-- in principle, write the state in some bases which look at the complicated sum. But they may be some other bases and be a simple product. So in general, it's actually hard to tell. In general, it's hard to tell, even though it's easy to say. But in general, it's hard to say.
So the entangled entropy-- so let me just call it EE just to save space. EE essentially provides a measure to quantify the entanglement between A and B-- because even in the case which you know this state is in entangled state, you may still want to ask how much they are entangled.
Entanglement entropy provides the way to quantify it. So the definitions are very simple. So first we look at this the density matrix for the total system. So if the system is in the state psi, then the density matrix for the total system would be just on the psi conjugate-- psi itself.
So in this basic matrix, we just trace out all the degrees of freedom in B. So since the Hilbert space have a tensor product structure, you can always do this. Then what you get is you get this the density matrix. And then here, you get a density matrix which only depends on the degree of freedom in A.
And then we just calculate the Von Neumann entropy corresponding to this row A. So entangled entropy is just defined to be [INAUDIBLE] entropy associates resistance in the matrix rho A. So this rho A is often called the reduced density matrix. And the Von Neumann entropy associated with the reduced density matrix is defined as the entanglement entropy. OK?
So this provides a very good the measure because say if SA is equal to 0, from our knowledge of the Von Neumann entropy, you can immediately deduce where the SA is equal to 0 if only if the rhos A, this density matrix is a pure state. It's a pure state. And then from here, you can also deduce rho A is a pure state. This reduced density matrix comes from this procedure as a pure state. Only if the psi can be written as a simple product.
So this tells you as when SA is non-zero, then you can be sure this state must be non-entangled. So whenever SA is equal to 0, you can be sure this is non-entangled. When SA is not equal to 0, you know for sure A and B must be entangled in this state. So that's why this is a good measure. OK?
And then the value of SA then will tell you how entangled the system is. And also, you may immediately ask the question what happens instead of defining the rho A? Of course, you can also do the same thing. You trace out A to define out rho B and then the define the entropy for the rho B. OK? Define entropy for the rho B. But you can easily show for any pure state psi SA always equals to SB. So it doesn't matter which are symmetric. So it doesn't matter which one you're looking at.
So this is very easy to prove, essentially just following from something called a Schmidt decomposition. And essentially right to this state in terms of Schmidt decomposition between the degrees of freedom rho A and B. And then you can show that rho A and rho B essentially have the same eigenvalues. And if rho A and rho B have the same eigenvalues, of course, then the entropy will be the same, because the entropy only depends on the eigenvalues. OK?
Any questions about this? Good. So here is what a mixed state-- about a pure state, let me just say a side remark. So if AB, the total system is in a mixed state-- so far always is in the pure state. But suppose it's in the mixed state. Suppose the system itself is described by a density matrix. So the total system itself is spread.
In general, SA is not equal to SB. OK? Not only in general, it's not equal to-- SA is not equal to SB. And in such a case, the entanglement entropy also contains classical statistical correlations of the mixed state-- of the mixed state-- so in addition to quantum correlations.
It's almost trivial to see because the people suppose here is not a pure state. So here you replace it by the density matrix. And when you trace all the B in this density matrix, then, of course, there's still some original uncertainty in the previous density matrix remaining in A. And then they will come into this entropy-- so as defined, will depend on the classical uncertainties-- classical statistical uncertainties of your original density matrix.
So for a mixed state-- so that's why for a mixed state, the internal entropy is not a very good measure of quantum entanglements, because it's contaminated by classical statistical information. But for today, we all need to talk about the pure-- mostly talk about the pure. Yeah, we actually talk about in general. But I want you to keep this in mind.
So any questions so far? So just to give you an a little bit more intuition, let's look at this very simple example to calculate entangled entropy. So let's consider a two-spin system. So let's consider you have two spins, OK? So this is my A and B. So this has a two-dimensional Hilbert space. This has a two-dimensional Hilbert space. Altogether, you have a four-dimensional Hilbert space.
So for example, so let me consider such a state. So this looks like a complicated state. But actually, this can be written as a single product, because you it can be written at OK, I hope this notation is for me. It's OK with you. You just A and B-- A spin and B spin.
So even though in this space, this is written as say a sum of state. It looks the entangleds. But in fact, it's not because you can write it in terms of a simple product. So this is another entangled state. Of course, for the simple system, it's very easy to tell. But to give you a complicated system with many, many, degrees of freedom, then it becomes very hard. And then entangled entropy becomes useful.
So now let me give you an example to calculated entangled entropy. So let's consider a state like this. So let's see some parameter. So clearly this state is entangled because you cannot write it as a simple product. So now, let's check it. Now, let's check it.
So you can look at the full density matrix of the system. You just look at this-- the bra and the ket itself. And then just do the product. And you get the quotient theta-- under the [INAUDIBLE] terms.
So you just take the product with itself. And then let's try to find what's rho A. So you trace out degrees of freedom of B. So this is our B, the second spin. And now let's trace out degrees of freedom B-- trace out the second spin-- so rho A. Goes one into we trace out the B in here. So when you trace out the B, you just take the end product between these two. So these are the same. So this will remain. And so you have this. And similarly, this one you have that. These two are the same. So when you take the trace, this is nonzero.
But this too will give you 0, because this one is orthogonal to this one. And this spin is orthogonal to that spin. So that's what you get. And then now you can usually just write down the entropy. So this is the entangled entropy for these two states-- for this two spin system as a general function of this parameter theta.
So now let's plot these as a function of S theta. So clearly, this is a period function. We only need to go to pi over 2. And then you have something like this. And you can easily plot that function. You will see something like this. So when theta is equal to 0. This is equal to 0 because this term is 0. This term is also 0 because of the quotient theta square is equal to 1. When theta equals to pi over 2, this term becomes 0 and this term also becomes, because it's [INAUDIBLE] 0.
But this maximum in the pi over 2 or pi over 4. So I had a pi over 4. Of course, you can also go to the pi equals to minus 4. OK, you can go do plus minus 4. I didn't go to the [INAUDIBLE]. Yeah, anyway, so these are called the maximum entangled states. This is a maximum entangled.
So this is a state in which you have the highest entropy. Any questions so far?
AUDIENCE: You switched it. It should be up down. It's down.
HONG LIU: Right, that's right. OK, good. So let me also say a few things about the properties of the entanglement entropy. So there are many properties you can derive from here. Let me only say a few important ones.
OK, so one property of the entangled entropy is called subadditivity condition. So if you can see that the two systems A and B, then you can show that S(AB), the entropy for the total system, is smaller than the sum of the separate system.
So when I write AB, I mean the combined system. OK? And this is greater than the difference between them. So this is so-called the subadditive condition. And then intuitively, you can understand. So if you have entropy of A and entropy of B, you add them together, then it's larger than the entropy of AB because there is some redundancy. There might be some redundancy here. Yeah, because when you add AB together, there may be some common correlation between them. And so this is greater than that.
OK, intuitively that's what this inequality means. And also there are some property for the strong subadditivity condition.
AUDIENCE: I don't quite understand--
HONG LIU: Yes?
AUDIENCE: What S of A and S of B means?
HONG LIU: So this is the entropy for the A. This is the entropy for the B.
AUDIENCE: Don't we need this to partition system A into two subsystems?
HONG LIU: No, you don't-- let me explain my notation. So S(A) means the entropy equals 1 if you integrate out everything else except A. And S(B) means you integrate everything else except B. And S(AB) be means to integrate everything else except A and B. And this is S(AB).
AUDIENCE: But in our case, A and B is all that there is.
HONG LIU: No, no, no. Now I'm just doing generally. Once I have this definition, so this can be-- even AB is a total-- yeah, so this can apply both to the case I said earlier-- say if you divide-- here I just-- so this AB does not have to be the same as that AB. Here I'm just talking about only two subsystems.
AUDIENCE: So it's for example, S(A) is like-- A and the supplement as two parts of the system. And they could have some entanglement. S(A) is just an entanglement field.
HONG LIU: A, S(A) is the entanglement of A with the rest. And S(B) is the entanglement of B between B and the rest. And the S(AB) is entangled between the AB together with the rest. Yes.
AUDIENCE: So to clarify one thing, when you say S of A, we have this whole system. It means that trace everything which is not A?
HONG LIU: Yeah, that's right. Any more questions about this? So here when I write to those expressions, I assume A and B don't have interceptions, OK? I assume A and B don't have interceptions. You can also have the strong subbadditivity condition. So this is pretty easy to prove. If we have time, it takes five minutes to prove.
But the strong subadditivity which I'm going to write down. So strong additivity is involving three systems. Now, you have to add the three systems. And then greater than ABC-- OK?
So, again, intuitively, the meaning of that inequality is clear. So the first inequality just says that I have two systems here. And the sum of these two systems-- the entropy of these two systems-- is greater than the sum between the combined system and the intersection between the two systems, OK, because C in the section between the two. And ABC is the whole thing combined.
And similarly here, he said they have S(A) and S(B). I have two A and B. So I trace out the system. Outside A, I get entropy for A. I trace the system outside B, anyway for B. This inequality says if now you attach A C to A and C to B, a common system to both A and B. And then the resulting system will be larger than the B form. So if you add something, we increase the entropy. That, of course, is intuitively clear, because if you have entropy essentially parameterized the unknown part of the system.
And if you add the third system, and then this just increase your unknown and then increase your entropy. So this strong subadditivity condition is actually very hard to prove. It's very hard to prove. It can get rather mathematical and requires some effort to do it. But this one is pretty easy to do. Yes?
AUDIENCE: Can [INAUDIBLE] about the first of the two equations? For strong subadditivity, what's the first equation?
HONG LIU: Yeah, so this means-- so look at this as a single system. This is a single system. That means that the sum of these two-- the entropy of this two system is greater than the sum of the combined system under the intersection. Any questions about this? Good.
So this concludes the very simple introduction to the entangled entropy.
AUDIENCE: Does the first one have any implications of topology?
HONG LIU: Sorry, what do you mean by topology?
AUDIENCE: Because it's like the intersection of the combined thing.
HONG LIU: Yeah, so as classical entropy, this is a very simple thing. You can easily convince yourself if ABC are classical systems, and just classical distributions, describe classical probabilities, statistical distributions. And then with those things, you can understand this inequality just by drawing this kind of standard diagram corresponding to the different sets.
The quantum mechanics proving those things are actually not trivial are not trivial. Quantum mechanically, they no longer come in a very intuitive way. Yes?
AUDIENCE: I was noticing something curious about the last line classically. If A and B are the same, I think the equation will still hold. But quantumly--
HONG LIU: It still holds.
AUDIENCE: Doesn't it not hold quantumly?
HONG LIU: No, they are supposed to hold quantum mechanically.
AUDIENCE: But we are under the assumption that A, B, and C-- they don't overlap, right? I'm saying if A and B are totally overlapping.
HONG LIU: No, but this inequality I'm writing in a way which they are not intersecting. You can write them in a way which they intersect. And I'm just writing in this way. So the condition for this particular form ABC is not supposed to intersect.
AUDIENCE: Right, I'm saying if we set A equals to B.
HONG LIU: No, no, then you cannot use this equation. Then you have to write the equation in somewhat different way, which apply to the intersection case. You can do them just choosing to write in the way which did only intersect.
AUDIENCE: OK, but the thing I want to say is that we can still keep the bottom equation classically, even if we took--
HONG LIU: No, both are applied classically. Both are trivial classically. You can understand very easily classically. It's just quantum mechanically, it's no longer trivial. Quantum mechanics is no longer trivial. And there are various ways you can write those equations. I'm just choosing to write the way which ABC don't intersect. And you can also write the way, just rename them so that they intersect.
AUDIENCE: So [INAUDIBLE]. So this is true for any entanglement state we choose?
HONG LIU: Any state, yeah, density matrix. Whether it's a pure state or density matrix, it doesn't matter-- a general statement. OK, good. So this concludes the short introduction to the entangled entropy itself. And you may know that the entanglement entropy actually plays a very important role. Entanglement and entanglement entropy itself plays a very important role in quantum information and quantum computing because of quantum entanglement is the kind of quantum correlations which you don't have classically. So this EPR paradox and the [INAUDIBLE] inequality, and the teleportation-- all those things that take advantage of.
But that's not our main point here. So what I'm going to talk about next is actually entangled entropy is also starting playing a very important role in our understanding of many-body physics and the space time.
So now, let's talk about the entanglement entropy in many-body systems in quantum many-body systems. So I hope you're familiar with this word many-body systems. It's just a system of a large number of particles or a large number of constituents. So quantum field theory issue is a many-bodied system. Any quantum field theory is a many-body system. But this also includes many other lattice systems, which condensed matter people use, which are not necessary quantum fields-- can be written as a quantum field theory. OK?
So now let's again consider just a simplified case. Let's consider a system which is composed of A and B-- so A plus B. And then I will say some trivial statements. So if H is HA plus HB. So now we talk about Hamiltonian. So far, I'm not using actually many-body systems. What I'm talking about are pretty generic systems. So even the Hamiltonian is actually just a direct sum of the Hamiltonian for A and Hamiltonian for B.
If they don't couple, then of course, the [INAUDIBLE] state or, in particular, the ground state is unentangled. So you just find the ground state of each system. And then that's the ground state of the total system. And also, in general, if you start with the initial stage, which is unentangled, with the initial state, which is unentangled, it will remain unentangled.
So if you started with an unentangled state, you wove it using this Hamiltonian, of course, it will just act on the specific part. And then you will remain unentangled. OK? And now let's consider we have H equals to HA plus HB. But now they also have interactions between the A and the B. So A and B are coupled.
Then in general, we can just repeat what he said here. Then the ground state is now entangled. OK, and now if you start with the initial state, even if you start with the initial unentangled state, it becomes entangled on Hamiltonian evolution.
So in general, we will expect-- so just based on this general expectation-- so you would expect the ground state of a typical many-body system will be actually rather entangled. It will be rather entangled unless the Hamiltonian factor rides into all three particles. The factor rides into the Hilbert space at each point-- for each degree of freedom.
But now let's talk about the many-body systems we're interested in. So they're either typical condensed matter systems or quantum field series. So in typical condensed matter systems, we face this problem. So in typical generic condensed matter systems of quantum field theory, in general, no matter how you divide A and B-- so generic H AB is non-zero.
But not only is H AB is non-zero, it's in general, H AB-- so the whole Hamiltonian, including H AB is local. This is a very important concept. It's local. But local will mean the following. For example, let me give you an example. For example, one of the very important condensed matter systems is, of course, the Heisenberg model. So essentially, you have the Hamiltonian.
So you can see the lattice in whatever dimension you're interested in whatever kind of lattice you are. At each lattice, there is a spin. So consider you have a lattice of spins. And then you'll have nearest neighbor interactions between the neighboring spins, so some of the nearest neighbors.
So you can imagine you have a lattice system. And each point on the lattice, you have a spin. And each spin interacts only with the spins nearby. So this is a local interaction. So by local interaction, means that the only direct couple to the spin falls distance away from it.
So this is an example of a local system. And all our QFTs are local systems. All our QFTs are local systems. For example, let me just write down the phi 4 theory, for example, phi 4 theory. Then phi at each point-- so now you have to go back to your first day of your quantum field theory where you emphasize locality. So the phi evaluated at each point now is independent of degrees of freedom.
Under the quantum field theory, it's a Hilbert space of a tensor product of the degrees of freedom. OK? Under the form of the Lagrangian say if you discretize it-- and this is only involving coupling between the neighboring point, because the derivative is only dependent on the second derivative.
And this only depends on the value of phi at the single point. So the typical quantum field theory as far as you have found a number of derivatives, it's all local. It's all OK intact. It's all local. OK? So in other words, in these kind of systems, let me go back here. So this is very important. In other words, in these kind of systems, so let me just imagine-- so let me just say this box is the whole thing. It's the whole whatever space I live in. And I divide it into A B.
So this tells you that for typical condensed matter or quantum field series, the H AB is only supported. So let me call this epsilon. So that tells that H AB is only supported near the boundary between A and B, because there is only local interactions. So the part of the Hamiltonian which directly covers A and B is only within this part. And this epsilon is added on the lattice spacing is order of lattice spacing, which in the case of a lattice system or in a quantum field theory, it would be your short distance cutoff.
So if you try to discretize your field theory, then this will be a short distance cutoff. So similarly, I can consider another shape of A. So suppose I can see the circular A. And then, again, the part of which H AB is only supported in the region between these two dashed lines. And those dashed would be considered to be very close to the A. I'm just going to pick. But this should be the short distance cutoff. OK?
And again, the H AB is only supported in here. So this is an important feature of which you have a local Hamiltonian. Let me just write one more word. So H AB only involves degrees of freedom here-- the boundary of A. So this means the boundary of A.
So this turns out to have very, very important implications-- the fact that the Hamiltonian is local. And that they only direct and mediate coupling between the degrees of freedom near the boundary of A. And they have very important consequences. So now I'm just telling you the result which is a result of having accumulated for many, many years since the early '90s.
So then when I find in the ground state of a local Hamiltonian in general you may construct some kind of evil counterexamples. But in general, in the ground state of a local Hamiltonian-- in the ground state of a local Hamiltonian-- any just choose any region A-- you integrate out the degrees of freedom outside of it.
So let me emphasize an important point. Let me just pause to emphasize an important, which is only increasing what I'm saying here. In this definition of the entangled matrix which I just erased, you just need to have a partition of a degree of freedom between A and B. The way you partition it does not matter. How you partition it does not matter.
You can partition it in whatever way you want. But for typical this kind of lattice system or for quantum field theory, there's a very large partition. And the partition is just based on the locality. It's just based on the degrees of freedom at each point. You just factorize your Hilbert space in terms of degrees of freedom at each point. So of course in the lattice system, it's obvious. You have a spin at each lattice. And for the quantum field theory, you just factorize your degrees of freedom. At each point, you factorize them. And so this locality provides a natural partition of your degrees of freedom.
And when we talk about A and the B, we always talk about in terms of the space location. And it's natural to talk about the partition in terms of just your metric locations. So is this point clear? I just want to emphasize this point. So then you find that in general for the ground state of a local Hamiltonian satisfy so-called area law is that the leading term of the entanglement entropy is given by the area of the boundary of A-- this applies no matter which dimension you are in generic dimensions-- and divide it by a short distance cutoff of the lattice spacing. It's some number.
So A is a dimensionless number. So area-- we can see the d-dimensional theory-- so d-dimensional system. Then the spatial dimension will be d minus 1. And then you go to the boundary of some region. The boundary of region A. Then that will be a d minus 2 dimensional surface. And then this would be the area of that surface. So this may cover a dimensionless number. And then you can have some pre-factor here which depend on your systems.
So this formula tells you a very important physics. So this formula tells you a very important physics. This tells you that generically if you-- in such kind of systems, AB are entangled. But the entanglement between AB between A is complementary, which I just call A and B, are dominated by short-range entanglements near the boundary of A where this H AB is supported.
OK? So this is also very intuitive once you load the answer-- once you load the answer, because we emphasized that H AB only coupled to degrees of freedom nearby. And then we look at the ground state you find most of the degrees of freedom in here don't entangle with B. Only the degrees of freedom them near the boundary of A are entangled with B because of this interaction. This local interaction directly leads to the entanglement between the degrees of freedom nearby. And those degrees of freedom, if they are far away, they might be entangled. But they are not dominant. OK? So I have put many dots here. So included in those dots are possible long-range entanglements. Because when you find the ground state, you really have to extremize the energy of the total system. You don't only look at the small part.
So there's always some kind of long-range entanglement beyond this H AB. But this formula tells you that this short distance entanglement dominates. Yes?
AUDIENCE: One question-- in QFT in general, like [INAUDIBLE], there's really all the interactions are perfectly local, I believe. But in string theory, are there any non-local interactions that occur. Are there any interactions with non-local?
HONG LIU: Yeah, it will depend on the scale. In string theory when we say non-local, it's non-local at the alpha prime scale. And that alpha prime can perfectly be our short distance cutoff here.
When I talk about local and non-local, I'm talking about infinite versus finite. For example, here, when I talk about whether this is a local system, I don't have to have a nearest neighbor. As far as this matches this point only directly coupled to the finite distance neighbor. And this can see the local Hamiltonian. But from the quantum field theory point of view, this might be considered non-local. You see what I mean? Depending on your scale.
AUDIENCE: So is there anything that appears in string theory which is non-local at any scale? Or is the non-locality just some--
HONG LIU: Yeah, so the string theory itself does not give you that non-locality, but the black hole may.
AUDIENCE: Oh, interesting.
HONG LIU: The black hole seems to give you some kind of non-locality which we don't fully understand. So that's why the black hole is so puzzling. And the [INAUDIBLE] of string theory, you're non-local in the scale of alpha prime. Yeah, if it's alpha prime sufficient small, it's like a local theory. And even if alpha prime is big, if you look at very much, it is still like local.
AUDIENCE: I see.
HONG LIU: Yeah, but black hole can make things very long distance. So that's the tricky thing about black holes. OK, so this is something which are is interesting and was first discovered in the early '90s. But in hindsight, it's very intuitive. In hindsight, it's very intuitive. So even though this formula, even though this term is universal, but this coefficient is actually highly non-universal.
Normally, by saying universal, we mean something which is common to different systems. So this coefficient actually depends on the details of individual systems. Say if you calculate for the Heisenberg model, or if you calculate for the free scale of field theory, or free fermion theory, and then this coefficient in general is different, are depending on how you define your cutoff.
So even though this formula is universal, but those pre-factors actually are highly non-universal. But the partial excitement-- there are many things people are excited about in terms of entropy. This is one thing, this area law, because the area law is nice. Then this can be used as a distinguishing property of a ground state.
If you go to a highly excited state, then you find the generic distribution inside and A and B will be entangled. And [INAUDIBLE] highly excited. But only in the ground state, somehow only near the boundary they are entangled. This is also makes sense, because if it were high energy then you can excite it, because from the very far away from H AB, then of course, everything would be entangled.
AUDIENCE: So just one other question. So why are you dividing by epsilon?
HONG LIU: No, this estimation is a number. Making the dimension is numbered. This is only a short-distance information here. So that is spatial short-distance cut off.
AUDIENCE: Right, the only reason I ask is that intuitively it seems that the entropy should be proportional to this thin volume around the boundary. But that doesn't-- OK, I don't know.
HONG LIU: Yeah, you have to make it into a dimensionless number. You have to be proportional to the area. So there's a very simple way to understand that thing. So the area divided by lattice spacing-- so what is this guy? This is wonderfully a lattice volume. That is the lattice area on the surface. So essentially this tells you how many degrees of freedom are lying on that boundary.
AUDIENCE: OK, interesting.
HONG LIU: Yeah, OK? So is this clear? OK, I think I'm spending too much time on this. Anyway, so excited in the last decade-- the main excitement about entangled entropy in the last decade. The one thing is about this behavior, then you can use it as a characterization of the ground state. But a lot of the excitement about this thing is on those dot, dot, dots.
So people actually find that the subleading term-- some excitement. Yeah, I'm just saying-- from last decade people have discover that the subleading terms in entangled entropy other than this area term which come in from long-range entanglements. So this captures the short-range entanglement from the long-range entanglement can actually provide important characterizations of a system.
So let me just mention two examples. So it will be little bit fast because we are a little bit-- do you want a break for the last day?
AUDIENCE: Well, when you say it like that--
HONG LIU: OK, good. OK, thanks. So let me just quickly talk about two examples because I want to talk about the holographic case. So the first thing is something called the topological can be used to characterize so-called topological order in 2 plus 1 dimensions.
So since the '90s, mid '80s, and the '980s, people discovered that if you look at the typical gapped system-- so when we say a gapped system means that system between the ground state and the first excited state have a finite energy gap. So our general understanding of a gap system is that in the ground state because you have a finite gap, and there's sufficient low energies, you simply cannot excite anything. You have low energy because you have a finite gap to the first excited state. You cannot excite anything.
So essentially, when you look at the correlation functions, we can look at all the variables. They all only have short-range correlations. The cannot have long-range correlation, because there's nothing to propagate you to long distance. And because long distance requires massive degrees of freedom. And because you have a gap, you don't have such massive degrees of freedom.
So the typical conventional idea about the gap system is that all correlations should be short-ranged. And so this is a condition that meets them. But in the late '80s and early '90s, our colleague, Xiao-Gang Wen, he introduced this notion of a topological ordered system based on fractional quantum Hall effect.
So he actually reasoned that there can be gap systems even though I have a finite gap, but in the ground state actually contain long-range correlations-- nontrivial, long-range correlations. And those long-range correlations cannot be seen using the standard observables. So if you are using the standard observables-- say correlation functions of local operators, you don't see them. You only see the short-range correlations.
And those long-range correlations are topological in nature. You have to see it in some subtle ways. And then in the '80s, except in the late '80s, early '90s, he was trying to argue in some [INAUDIBLE] way to argue there is such kind of subtle correlations. But then around 2000, maybe 2005, then he realized actually you can see it very easily directly from the ground state wave function. You just calculate the entangled entropy. You just calculate entangled entropy.
So in entangled entropy, you always have this-- so this is a 2 plus 1 system, and then the boundary is just a line. So essentially, you just have a boundary. It's a number-- the length of the boundary divided by your cutoff, but then it turns out for these kind of topological ordered system, there is a finite constant as a subleading term.
And this finite constant is independent of the shape of A and independent of length of A and independent of whatever A is purely topological in nature. And so if you have achieved a gap system then this gamma will be 0. So you look at a free massive scale of field-- then this gamma will be 0. But for those topological ordered systems, this gamma would be non-zero. And it tells you actually there is long-range correlations which are encoded in the entanglement entropy, because this cannot come from the local thing because this does not depend on the shape of A, does not depend on N sub A, does not depend on anything of A. So this is really topological in nature. It tells you this can only be some long-range, subtle correlation, which is only capture by these entangled entropy rather than by the standard correlation functions. OK
So this is one discovery which is generated a lot of interest in the entangled entropy because now this can be used to define the phases. OK, you can actually define non-trivial phases using this number. And so this is the first thing. So this is about in condensed matter systems. In quantum field theories , this can be used to characterize the number of degrees of freedom of a QFT.
Again, this is a long story. But I don't have time. So let me just tell you a short story. So first let me look at 1 plus 1 dimensions, a CFT. So for 1 plus 1 dimension of CFT or important the quantity is so-called essential charge. So have you all heard of essential charge or not really?
It just says, imagine you have a 1 plus 1 dimensional CFT. And for each CFT, you can define a single number, which is called the center charge. And this center charge is very important because it controls the asymptotic density of state of the system. So if you look at the system go to very high energies, and the density of state essentially is controlled by this number C.
So essentially C can be used to characterize a number of degrees of freedom. And the C will appear in many other places. But I don't have time to explain. But anyway, there's something called the central charge, which can be used to characterize numbers of degrees of freedom of the system. And thus, if you look at the entanglement entropy for 1 plus 1 dimensional system for 1 plus 1 dimension of CFT. So in 1 plus 1 dimension, you only have a line in the spatial direction.
So then this takes A just to be a sacrament of length l. Then you find when you integrate out everything else, the entangled entropy for the A given by C divided by 3 log L divided by epsilon. Again, epsilon is a short distance cutoff. And the C is your center charge. So you see that the entanglement entropy-- first you notice that formula becomes degenerate when you go to the two dimensions.
It's equal to 1 plus 1 dimensions. 1 plus 1 dimension the were played by something like this. You have log epsilon. So the key important thing is not the prefactor because of the log. The prefactor is actually reversal. And it's actually controlled by the central charge. So that saves you from entangled entropy. You can actually read the central charge and actually read what are the numbers of degrees of was freedom of the system.
So the fact that the central charge in the 1 plus 1 dimensional CFT captures the number of degrees of freedom was known-- known before people thought about entangled entropy. But it turned out-- and people spent many years trying too hard to generalize this concept to higher dimensional series. And it turned out they are very hard to generalize. People didn't really know how to do it. But now we know because you can just generalize the entangled entropy to higher dimensions.
And then you find the same kind of central charge which appeared. You find again things can be defined as analog of the single charge in 1 plus 1 dimension appears in the entangled entropy in the higher dimensions. And then they can be used to characterize the number degrees of freedom. And so in some sense, the entangled entropy really provides a unified way to think about this central charge and to characterize the number of degrees of freedom across all dimensions-- across all dimensions.
And so this is a key formula. Actually, I first realized by our old friend Frank Wilczek when they studied free field theory. And people later generalized to show this actually works for any CFT.
Good. So now finally, we can talk about holographic entangled entropy with lots of preparations. So this just gives you some taste of actually why we are actually interested in entangled entropy and why this actually not only people in quantum computing or quantum information people are interested in it. People are doing condensed matter-- people doing quantum field theory are also interested in it.
And now people who are doing string theory are also interested in it because of this holographic entanglement entropy. So suppose we have our CFT. So a two-dimensional CFT with a gravity dual-- so a dual to some theory in d plus 1 dimensional [INAUDIBLE] space time. So let me give you origin A. How do I find the entangled-- what is the counterpart of this entangled entropy on the gravity side? So this is the question.
So let me just draw this figure. Suppose this is the box again that represents your total system. And then this carves out of region A. Then the question what does this translate into? And the graph decides. OK, so now in the boundary, we have some region A is equal to 0.
So for this question, in some sense it's difficult because it's not like other questions we've talked about before. So we also know partition function. You can say the partition function cannot be the same. Those things appear to be natural. Here, there is no natural thing you can think about, because you cannot define entangled entropy as a partition function. Entangled entropy have to involve in so-called trace out the degrees of freedom. Then take the logarithm. It's highly non-local and a complicated procedure.
And it's obvious how you would define on the gravity side-- how you would guess on the gravity side. So it's quite remarkable and the Ryu-Takayanagi, they just made the guess. And then worked.
So the proposal is just find the minimal area surface, let me call gamma A, which extends in the back and with the boundary of A as the boundary. You first find the surface. And then you say the entangled entropy for A is just the area of this gamma A divided by 40 Newton. And this is the Ryu-Takayanagi.
So the idea is that you find a surface of a minimal area is going into the back, but ending on the boundary of A. So this is your gamma A. And you find such a minimal surface. Then you divide by 40 Newton. OK? So let me just mention one thing.
Let me just note a simple scene, because again, the A of the boundary, this is d minus 2 dimensional. And A and the gamma A are all d minus 1 dimensional. So this is d minus 2 dimension is what we said before because A is just a region in your spatial section. So A is a d minus 1 dimensional. And the boundary A will d minus 2 dimensional. And the gamma A would be a surface which is ending on the boundary of A. This ends on that thing. So this is also the d minus 1 dimensional.
And this is d minus 1 dimensional. So this area have dimension d minus 1. And that's precisely the dimension of G Newton in the d plus 1 dimensional [INAUDIBLE] of space time. And then this is a dimensionless number. OK, because we did it the SA with dimensions. So you can say this is a very difficult guess. You can also say this is a very simple guess, because clearly this formula is motivated by the black hole entropy formula.
You may say, ah, black hole entropy is something divided 4GN. If it is entangled entropy, it's also entropy. Maybe it's also something divided by 4GN. And maybe it's some surface divided by 4GN. And then the special surface would be the minimal surface. So in some sense, it's a very naive guess. And you could say it's a very simple guess.
But as I said, it's also a very difficult guess, because essentially, other than that, you don't have other starting points. Yes?
AUDIENCE: So [INAUDIBLE]. So we have A in the CFT. Where do we know exactly where to place A in the AdS? Because it's not--
HONG LIU: This is a good question. This is what I'm going to say next. Yeah, but let me just emphasize that. So when we talk about A, we're always talking about the constant time slice, because you have to specify some time. And actually, when we consider the ground state or typical state does not depend on time. And it actually does not matter which slice we choose. You just choose a time slice. And then you specify region A, because to talk about degrees of freedom, you only talk about it in the spatial section.
So if you have a ground state or have any state which does not depend on time, then the gravity side also does not depend on time. It's a time-dependent geometry. So time slicing in the field series naturally extends in the gravity side. So in the gravity side, this surface would be in the constant time slicing, which would go to the gravity side.
AUDIENCE: Professor, one more thing. With the CFT and AdS-- I know we like to think one existing on the boundary of the other. But how does-- that's sort of an abstraction, right?
HONG LIU: No, that you should really consider as a real thing-- real stuff. Yeah, if you just think of that as an abstraction, then you miss a lot of intuition, because you use that as a genuine boundary, will give you a lot of intuition. And many things become very natural. Many things become very natural. Yes?
AUDIENCE: So comparing to the other formula that also has an area of the boundary, all this tells us is just it helps us count the number of degrees of freedom in the CFT. So it all it tells us is basically the epsilon in the denominator?
HONG LIU: No, no, they have a complete different dimension. No, this one higher dimension.
AUDIENCE: So that's another strange--
HONG LIU: No, this is not strange at all, because they're not supposed to be the same. No, this is the way it should be. So this is d minus 1 dimensional. And that is the d minus 2 dimensional. It's just completely different.
AUDIENCE: Well, right, but they are not-- there is something the same about them.
HONG LIU: No, there is something to same about them. But these two have completely different physics. So this has a close analog with black hole physics. Don't think they're motivated by that formula. I think they're motivated by the black hole formula. And people also try to connect that formula to the black hole formula. That's another story. Anyway, it doesn't matter.
OK, good. So this is a guess. Yes?
AUDIENCE: So is this result for the ground state on the CFT?
HONG LIU: No, this is valid. Yeah, for the time-independent state. The eigenstate is not invoked with time.
AUDIENCE: The thing I don't understand is on the site of the CFT, there is this state that you did as an input to calculate the entanglement entropy. And what's the corresponding thing on the AdS side?
HONG LIU: The AdS side is the corresponding geometry. So it's as said before, each state on the field theory should correspond to some geometry with normalizable modes. Yeah, so it's the corresponding geometry. And the ground state, then this will be just pure ideas. And if you look at the final temperature state, then this will be the black hole. And then if you are able to construct some other geometry corresponding to some other state, you can use that.
AUDIENCE: How about this entropy on independent on which state we choose for CFT?
HONG LIU: No, of course it depends. No this formula does not. But the geometry does. The geometry does. This is a minimal surface in whatever [INAUDIBLE] geometry.
AUDIENCE: Does the region-- after we choose the region, we can choose any state--
HONG LIU: Yes, yes.
AUDIENCE: And as a minimal surface, is it independent on which state we choose?
HONG LIU: Of course, it would depend on which state you choose, because each state corresponds to a different geometry. And the minimal surface depends on your geometry. So each state-- so remember previously, the state on the CFT side is mapped to the geometry with normalizable boundary conditions with normalizable modes. Yeah, anyway, just let me say just geometry.
So a state with the geometry. If you have a different state, you have a different geometry. But by definition, all this geometry would be asymptotic AdS. So in a boundary, they should all be asymptotic AdS. And so that what means to have [INAUDIBLE] mode excited. OK? Yes?
AUDIENCE: So this entropy formula takes into account the entropy of gravitational excitations in AdS, if you can put it that way. But black hole entropy--
HONG LIU: No, let's try not to jump too fast. And I think you are trying to extrapolate too fast. This formula is supposed to calculate the entangled entropy in the field series side in the [INAUDIBLE] limit and the notch lambda toward coupling limit. And we can talk about the gravitational interpretation later. But this formula is about the field series entanglement entropy. OK? Good. Any other questions? OK, good.
So there are many support of it. So this is a rule of the game. You make a guess. Then you do a check. If the check worked, then that gives you confidence, and then you do another check. And here you find it will fail. And if you do a sufficient number of checks, then people believed you. Then people will believe you. And everybody started checking it. And then sooner or later we will see whether this fails or works.
Anyway, so this is nice because this is simple to compute, because finding a minimal surface on the gravity side is in some sense, it's a straightforward a mathematical problem conceptually, even though technically it may not be simple. But it's straightforward conceptually. Anyway, so you can use these to calculate many quantities. Let me just say in support of this-- let me just say it in words just to save time.
So the first thing you should check is that this satisfies the subadditive condition which we wrote down earlier, because that's a very non-trivial condition. It should be satisfied by the entropy. So first, you should check that, because if you violate that, than this proposal is gone. And then there are other things that you can try to use to reproduce all the known behavior we know about entangled entropy, for example, this behavior and this behavior-- this area along.
And then we actually build up confidence by checking all those known results. And then you can try to derive [INAUDIBLE] and to see whether [INAUDIBLE] makes physical sense. So essentially, that's how it works.
And this is nice also at the tactical level, because entangled entropy is something very hard to compute. I don't know. You guys may not have experience of calculating entangled entropy. But ask Frank Wilczek. He was one of the first few people to do it in some free field theory. Even for free field theory, people could mostly do it in 1 plus 1 dimension. Going beyond 1 plus 1 dimension free scalar field theory in 2 plus 1 dimension 3 plus 1 dimension for some simple region like a circle or sphere you have to do a numerical calculation. You have to discretize the field theory to do very massive numerical calculation.
It's not easy to compute. It's not easy to compute. But this guy is actually easy to compute in comparison. So this guy at the technical level provides a huge convenience to calculate many things. Yeah, so this is a side remark.
So now let's just do some calculations. So first, let me show that this actually satisfies strong subaddivity. So remember, the formula we had before is S(AC), unfortunately I erased it. OK, so this is one of the inequalities. So we are just drawing it 1 plus 1 dimension because it's easy to draw. But a similar thing can be easily generalized in a higher dimension. So let's look at just the line in 1 plus 1 dimension. So this can call this region A. This is region C. This is region B.
You can also make them separate. It doesn't matter. You can also make them separate. It's easy to work out. So I'm just giving you one cases. So suppose a minimal service for the AC reading is like this. And the minimal surface the BC reading would be like this-- so minimal surface like that.
So let me call this curve gamma AC and this curve gamma BC. OK, I could not find the colored chalk today. So I did not have the colored chalk. Let me see whether this chalk works. Is this the colored chalk? Yes. So now, let me define two other surfaces. Let me call this one gamma 1 and this one gamma 2. In principle, I should use two different chalks for that.
You think it looks like something? OK, anyway, so we have gamma AC plus gamma BC equals to gamma 1 plus gamma 2 because-- gamma AC-- this is length-- gamma BC is equal to gamma 1 plus gamma 2. And then just from the definition of the minimal surface, then the gamma 1 must be greater than gamma C in terms of the length because the minimal surface associated with C must be the minimal area. It must be smaller than gamma 1. Under the area of gamma 2 must be greater than gamma ABC, because the gamma 2 is the boundary is the ABC. And the gamma 2 must be greater than gamma BC because this is supposed to be the minimal surface.
So now we conclude that gamma AC plus gamma BC is greater than gamma ABC plus gamma C. And then this translates into that formula. OK? Very simple, and elegant proof-- very simple and elegant proof. And now you can prove another inequality. You can prove another inequality, which is S(AC) plus S(BC) greater than S(A) and S(B).
So now, I will define another surface. OK? So now, let me just redraw it-- A, C, B. So I have AC like this. I have BC like this. And now, let me call this one gamma 1 tilde-- this one gamma 2 tilde. This is annoying. OK. And again, gamma AB plus gamma AC plus gamma BC equals to gamma 1 tilde plus gamma 2 tilde.
So the gamma 1 tilde ends in A. Gamma 2 tilde ends in B. That means gamma 1 tilde must be greater than gamma A and gamma 2 tilde must be greater than gamma B. OK? So now you show that gamma AC plus gamma BC is greater than gamma A plus gamma B. So this is very easy.
So this is a very simple and elegant. So if you want to really to be impressed by this, I urge you to look at the proof of the strong subaddivity itself in say in some textbook or in the original papers. It's actually highly non-trivial. You need some double, double concave functions. It's quite a height involved.
OK, so this is a great confidence boost that satisfies the strong subadditivity condition. So now, let's look at the last one. Let's try to reproduce this formula. Let's try to do a simple calculation to reproduce this formula. So this formula is supposed to be true for any CFT. This should be also applied for the holographic CFT and to check whether this formula works.
The reason you would like to choose an example which is new. But this example is the simplest. So that's the reason I choose this example, even though it's just reproduced all the results. So let's now look at the entangled entropy of 1 plus 1 dimensional CFT. So here, now you have a CFT 1 plus 1 should be dual to the AdS3. You will do some theory in AdS3.
So the S squared-- so let's only look at the vacuum then we just work with AdS3. So let me write down the AdS3 metric. So dx is the boundary directions. So as I said, each CFT is characterized by a central charge. And you can obtain a central charge from various different ways. And again, from the holographic, you can try to express the single charge in terms of gravity quantities.
And there is many ways to derive it that we will not go through with that calculation. So let me just write down the results. So far the holographic CFTs, the single charge is related to the gravity quantity by 3R divided by 2 G Newton. So this is a result which I just caught. I will not derive it for you. But you can derive it in many different ways.
So for CFT, to do a gravity idea 3, its central charge is given by 3R, which is the AdS radius divided by 2 times the Newton constant. And the Newton constant in three dimensions has mass dimension 1. So this is a dimensionless number-- a dimensionless number. So now let's calculate the entanglement entropy using that formula.
So this now reduced your calculation very similar to our Wilson loop calculation. So let's call this L divided by 2. So lets call this X direction. So this is a V direction. So this is L divided by 2. This is minus L divided by 2. This region is A. Then we need to find a curve, because this now 1 plus 1 dimensions-- one dimensions. So we need to find the curve which end on this segment of A. OK? So is this clear?
So this is the x direction. And so this is 0, x equals to 0. Yes? OK. So what we need to find is find the minimal surface connecting these two points-- a minimal curve-- a minimal length curve connecting these two points.
So it's as we said before, we should look at the constant time slice because this is a vacuum which is time independent. Let's look at the constant time slice. The constant time slice, the metric becomes-- so in this space, the metric becomes r square divided by z square because the time does not change. So now if I treat the x as a function of z, and then these will become. So this curve lets me parametrize x as a function of z. Then this just becomes r square divided by z square 1 plus x prime square dz square.
And so this is dl square. So the length of the curve square would be just parametrized by this guy. OK? And we have to satisfy the boundary condition-- by symmetry, we only need to consider the right stuff OK, because it's symmetric. So we impose the boundary condition at the x z equal to 0. It's L divided by 2. We can see the right half. So now you can just write down the entanglement entropy now.
So S(A) will be 1 over 4GN. So I integrate half of the curve. Let me just call here z is equal to 0. Let me call this point z0. So I just integrate from 0 to z0. Z0 we will find out when you find out this minimal length curve. So this one will be r/z dz. Then just taking the square root of this guy-- the square of 1 over x prime square.
So this is the length and the times 2, because we only can see that this is half of it. And now you extremize it. Then you find the minimal length curve. And then you plug the solution here. Then you find the action. Actually we don't need to extremize it, because this is a well-known problem. And this is called the Poincare disk. So this is a prototype of a two-dimensional hyperbolic space. And the minimal length curve inside your hyperbolic space is maybe a 16th century problem. Yeah, I don't know. Actually, maybe 17th century.
Anyway, so the answer is actually very long. But you can also easily work out yourself. So the result is actually this is just exactly a circle. OK? Exactly a half circle. So that's actually a half circle. That means that x equals 2. The half circle with a radius L divided by 2. So this has just become L squared divided by 4 minus d square.
In particular, z0 corresponding to the point which x equal to 0 just L divided 2. So now you just plug this into here. You just plug this here. Let me just write it a little bit fast, because we are a little bit out of time. So you plug this in. Then you can scale the z out. You can scale z to be scaled L divided by 2 factors out. And let me just scale it. You can rewrite the integral as follows.
So after the scaling it's say 2z scale with L divided by 2z to do the scaling. Then you find this expression becomes 2r/4GN. And the upper limit becomes 1. And the lower limit you see. When you plug this in, actually the lower limit is divergent because there's 1 over z here. As always, we need to put the cutoff. And also, this divergence is expected because of below that here it depends on short distance cutoff it would be divergent.
So we need to for the short-distance cutoff here. So let me put it here epsilon. And this will be 2 epsilon divided by L when I do this scaling. And then you have dz/z 1 minus d square. So the integral reduces to something like this. And now you can evaluate this integral trivially. Then you find the epsilon goes to the limit.
Then you find the one term survives 2r divided by 4GN log L divided by epsilon. So now let's try to rewrite in terms of this C-- we rewrite in terms of C. So this is 1/3 3r divided by 2GN log L divided by epsilon. And so this is precisely that formula C divided by 3 log L of epsilon. And with that, you just count from the minimal surface in the hyperbolic space is a circle. And it takes a lot of effort to calculate this thing, even to do a free field series calculation. But in gravity you can do it very easily.
So now let me just quickly mention what happens if you do at a finite temperature. So you can also do this at a finite temperature. So since we're out of time, we'll be doing it a little bit fast. So first doing it at a finite temperature is easier. Let me just say finite T. And then I will connect to the black hole entropy. I will show that this formula actually reduces to the black hole entropy in some limits.
So for these problems, actually, it's easier to consider CFT on the circle. OK. So when the CFT is on the circle, the boundary will be a circle because this is 1 plus 1 dimension. So remember, in the global ideas, it's like sitting there in the boundary. And in the 1 plus 1 dimensional case and the boundary is just really a circle. And this is the bark
And a finite temperature, you put the black hole there. Let me just put the black hole here. OK? Just put the black hole there. So now you can ask the following question. So now let's consider some reaching A. So let's consider A is very small. Yo can work out the minimal surface. So it acts the same as our Wilson loop story. So you find a minimal surface. But if A is sufficiently small, then the geometry around here is still AdS.
And then you just get a minimal surface around here-- so a small deformation from the vacuum behavior. So we'll make A larger and larger. So this z0 depends on L. If we make L larger and larger, this is z0 will be deeper in the bark. So if you make A larger, so this will go more to the bark and then will be deformed, and then we'll see the black hole geometry.
But now to answer the question how about if I make A to be larger-- this region to be A to be larger than the half of the size of the circle. So the minimal surface should be able to be to be smoothly deformed back into A. So now you have to do something like this. It turns out the minimal surface want to go around the horizon and then doing something like this.
AUDIENCE: Why don't you do it the other way around?
HONG LIU: No, it's because the other won't. It's because A is like this. And if you do something like this, then it can be deformed back into A. It's mostly without crossing the black hole.
AUDIENCE: Yes, or it could be like a phase jump or something at some point when one surface becomes longer than [INAUDIBLE].
HONG LIU: No, in this situation, it's not that thing. It's something like this. Now, if you make A even longer, eventually, let's take the A even longer. And that would be something like this. Yeah, because you always want to hug near the horizon. And eventually, we'll make A to be tiny, then it will become something like this. You have something close on the horizon. Then you have something like this. It's roughly something like this. Yeah, is it clear to you? It doesn't matter.
[LAUGHTER]
So now let's consider-- anyway, yeah, let me just draw this again. It actually does matter. Sorry. So let's take A to be very small. Let's take A to be a very big. This part will be very small. So we are finally we will go like this. Anyway, something like this when you hug the black hole. And eventually, when these two points shrink, so take A with the total region. Take A to be the total space. What do you get? Then what you see that the minimal surface essentially is just essentially a surface hugger on the horizon.
And this is a precise black hole formula, because then this is a imprecise a black hole formula. And this is a situation you expect it to be black hole formula, because if you take the A to be the host space, then rho A is just equal to the rho of the whole system. And the S(A) by definition is just equal to the S of the whole system. And this is just a thermal density, a thermal entropy.
And this is given by the black hole formula. It's given by the black hole formula. And this is given by [INAUDIBLE]. So you find in these special cases, actually, you recover the black hole formula.
So I have some small things. We can talk a little bit more. But I think we'll skip it. You can also show that actually for general dimensions, you always recover the area more without the details of the geometry, because no matter what kind of dimension you look at, the typical state which will be normalizable to geometry.
When you approach the AdS-- when you approach the boundary, it's always like AdS. And then you find in a higher dimension, it's always like this. It says, when this minimal surface is close to the boundary, it becomes perpendicular to the boundary. Here is a circle, then of course, it's perpendicular to the boundary.
But you see this feature is actually generalized to higher dimensions-- to any dimensions. So what you see is that in the general dimension, a minimal surface will be just near the boundary will be just perpendicular to going down from everywhere. And then from there, you can show that you always have the area law from this behavior. You always have the area law. So this can give you an exercise for yourself.
Yeah, so let me just finish by saying a couple of philosophical remarks, and then we'll finish. So this RT formula is really remarkable, because it's actually, as I said, technically provides a very simple way to calculate entangled entropy. But actually, conceptually, it's even more profound because it tells you that the space time is related to the entanglement, because it simulates something which is very subtle from the field theory side, because this S(A), as we said, you have to do some complicated procedure to trace out degrees of freedom-- take the log.
And that turns out to be related to the geometry in a very simple way. So essentially, you see that entanglement-- it's just captured by the space time. And in particular, it's something you can say the geometry is essentially equal to the quantum information. So this is a very exciting also from the gravity perspective, because if they ask you if you really understand this duality, then you can actually using the quantum information of the field theory to understand the subtle things about the geometry. It just tells you the geometry is actually equal to the quantum information.
So for many years, actually not that many years, so previously, we have people doing quantum information. And we have people doing this matter, which is the quantum many-body system. And this matter, or QCD, so this is kind of quantum many-body. And then we have people doing black hole, string theory, and the gravity.
So they are all very different fields. But now, they're all connected by this holographic duality. Somehow essentially, they connect all of them. So now, we essentially just see a unified picture, a unified paradigm, to understand all quantum systems, including quantum systems without gravity, with gravity. So I will stop here.
[APPLAUSE]
Free Downloads
Video
- iTunes U (MP4 - 259MB)
- Internet Archive (MP4 - 259MB)
Subtitle
- English - US (SRT)