Flash and JavaScript are required for this feature.
Download the video from iTunes U or the Internet Archive.
Description: In this lecture, Prof. Liu first discusses important scales in classical and quantum gravity, and then reviews the spacetime geometry of a black hole.
Instructor: Hong Liu
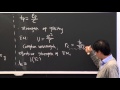
Lecture 2: Classical Black ...
The following content is provided under a Creative Commons license. Your support will help MIT OpenCourseWare continue to offer high quality educational resources for free. To make a donation, or to view additional materials from hundreds of MIT courses, visit MIT OpenCourseWare at ocw.mit.edu.
PROFESSOR: OK, let us start. So let me first remind you what we did in last lecture. We proved-- we showed that the Weinberg-Witten theorem for beats existence of massless spin-2 particles. Massless spin-2 particles are hallmark of gravity, so that's why we look for them. In the same-- so emphasize in the same space time, say a QFT lives.
So this theorem essentially cites this. You will never have emergent gravity start from a [INAUDIBLE] quantum field theory, which is a well-defined stress tensor. So as we already mentioned, it's a new pole of this theorem is that actually emergent gravity can actually live in a different space time. So as in a holographic duality. In fact, in holographic duality the gravity lives in one dimension higher, but we are not ready to go there yet.
So in order to describe this thing we still need to do some preparations. So the preparations-- let me just outline the preparations we need to do. So the first thing we will do is black hole thermodynamics. So these will give hint for something called the holographic principle, which is actually more general than the holographic duality discovered so far.
And the second thing is, we will also quickly go over the large N gauge theory-- the properties of the large N gauge theories. So this give hints-- something called a gauge string duality. So the behavior of the large N gauge theory give you a strong hint that actually there's a string theory description for ordinary gauge theories.
OK, and then when you combine these two things together, then you get what we have currently, the holographic duality. And then we will also talk a little bit of sting theory. A tiny bit. Not a lot, so don't be scared. So we also will talk a little bit about string theory. So in principle, actually, I can talk about holographic duality right now, but going over those aspect can help you to build some intuitions, and also to have a broader perspective than just presenting the duality directly.
So before I start today's lecture, do you have any questions regarding our last lecture and regarding this-- yeah, general remarks here? Yes?
AUDIENCE: So moving forward, are we going to define emergent gravity as this existence of a massless spin-2 particle?
PROFESSOR: No, that we will not do.
AUDIENCE: So how are we defining emergent gravity?
PROFESSOR: You construct Newton's Law? Yeah, you will see it. You will see it when we have it. Yeah, essentially you see-- essentially it's handed over to you. You don't have to go through that step. You don't have to go through that step. Yeah. Any other questions? OK, good, let's start with black hole thermodynamics.
So let me start by doing a little bit of dimensional analysis, just to remind you important scales for the gravity. So the first thing is what we called the Planck scale. So in nature we have fundamental constant H-bar, Newton constant, and the C, speed of light. And immediately after Planck himself introduced this H-bar, he realized you can actually combine the three of them to come up with a map scale, which is just hc divided by GN. And this, if you plug in the expressed numerical value, it's about 1.2 to the 10, to the 19 gev, divided by c squared.
You can also write it in terms of the gram, which is about 2.2 10 to the minus 5 gram. And then you can also have-- we can also construct the length scale, which is hGN divide c cubed, which is about 1.6 to 10 the minus 33 centimeter. And tp equals lp divided by c, OK?
So this was discovered-- Planck introduced them in 1899, so most of you may know the story. And then, just immediately after he introduced H-bar-- so he introduced H-bar in 1899. In the same year he realized you can write down those numbers. And of course, those numbers meant nothing to him because at that time he didn't know special relativity, he didn't know quantum mechanics-- essentially, he didn't know anything.
But he famously said that this unit-- so he claimed those should be basic units of physics, and he also said these are the units that would retain their significance for all times and all cultures. He even said they even will apply to aliens. But only after 50 years-- so after 1950s people get some sense. So many years after special relativity, many years after general relativity, and also quantum mechanics, et cetera, people started grasping the meaning of those scales.
And so let me briefly review them. So you can-- OK, the feeling of the meaning of those scales by looking at the strength of gravity. So let me first start this example for electromagnetism, which you have a potential, which is e squared divided by r. So if you have two charged particle of charge e, then the potential between them is e squared divided by r.
And then for particle of mass m then you also have Compton wavelengths. So if you have a particle with mass m, you also have Compton wavelengths. It's H-bar divided my mc. So you can get rough measure of the strength of electromagnetism-- the actual strength of the electromagnetism by conceiving the following [INAUDIBLE] dimension number, which I call lambda e, which is the potential evaluated at the minimal-- in quantum mechanics, essentially this is the minimal distance you can make sense of. Because once you go distance smaller than these, you can no longer-- and then the quantum uncertainty will create the energy uncertainty bigger than m, and you can no longer talk about single particle in a sensible way.
So the essentially the minimal length scale you can talk about single particles. So this is essentially some energy scale. So you can compare these to the, say, the static mass of the particle. So this give you a measure of the strength of electromagnetism. Of course, if you plug this in you just get e squared divided by H-bar c. And of course, we know this is the fine structure constant, which is indeed the coupling. Say you would do-- indeed, it's a coupling in QED, OK?
So you can do the same thing for gravity. You can do the same thing for gravity. So for gravity we have essentially g, and say if you take two particle of masses m then the potential between them is gm, m squared, divided by r. And then again, you can define effective strength for the gravity. I evaluate this potential at Compton wavelengths divided by the static energy of the particle. And then you can just plug this in.
So this is GN m squared, divided by H-bar, divided by mc, then divided by mc squared. And then you find this is just equal to GN m squared, divided by H-bar c. OK, so now if you compare with this equation-- OK, so this is just given by m squared divided by mp squared. OK, so for gravity this effective strength is just given by the mass of the particle. So because for gravity the mass is like some kind of effective charge, and then divided by Planck scale-- this Planck mass-- squared.
OK, and you can also write it as lp squared divided by rc squared, or just as this Planck length divided by [INAUDIBLE] wavelengths of this particle. OK, so for most elementary particles-- so for typical elementary particles we know the m is always much smaller than mp. And then the lambda g would be typically much smaller than 1. So for example, say, if you can see the electron, the electron mass would be 5 to the 10 to the minus 4 gev divided by c squared.
Of course this is much smaller than this Planck mass. And then you find that this ratio-- so you can work out this ratio. So let's compare it to the corresponding strength for electromagnetism. So this is about 10 to the minus 43 if you work this out. So this tells you the gravity's really weak. So for ordinary elementary particles-- so the gravity is really weak, and so we can forget all about gravity until you reach the Planck mass, or your Compton wavelengths reach the Planck length. And then the fact of the quantum gravity will be important.
So from this exercise we know that the mp is the energy scale, that the effective gravity strength become of order one-- become of order one-- that is, quantum gravity fact becomes significant. And similarly-- so lp is the corresponding length scale associated with such energy. So this give you a heuristic feeling-- give you a heuristic indication that the meaning of those Planck scales.
OK, so there's another important scale associated with gravity. So any questions on this? Good, there's another important scale associated with gravity. It's called Schwarzschild radius-- Schwarzschild radius. So just from dimensional analysis, the Schwarzschild radius can be argued as follows. So can see that-- again, we can just even see from Newtonian gravity. So I would say let's consider the object of mass m. Then we ask is the distance-- at what distance, maybe I should-- at what distance from it the classical gravity becomes strong.
So for this purpose, let's consider, say, a probe mass-- say, m prime. So, can see the probe mass. So I define a scale which I call rs, as I require the potential energy between m and m prime. at such a scale rs, then this become of order, say again, the static energy of this probe political, or of this probe mass. So if you cancel things out then you find rs is of order GNm divided by c squared.
OK, so this give you-- I'll give you a scale. You can also ask-- so this is from Newtonian gravity. Of course, when your gravity becomes strong you should replace the Newtonian gravity by Einstein, the relativity. And then when you go to relativity-- when you go to relativity, general relativity, then you find then there's a Schwarzschild radius. So there's a Schwarzschild radius which given by 2 gm divided by c squared, which corresponding to the sides of a black hole. OK, corresponding to the sides of a black hole.
So this is a classical scale-- purely classical scale. Just the scale which the classical gravity becomes strong. In particular, rs can be considered as the minimal length scale one can probe an object of mass m, OK? So classically, black hole absorb everything. So once you fall into black hole you can never come back. And so the minimal distance you can approach an object of mass m, it is given by the Schwarzschild radius. So when you go inside the Schwarzschild radius we just fall into the black hole, and you can never send information out.
And the one interesting thing compare-- yeah, one interesting thing regarding this Schwarzschild radius. You said Schwarzschild radius increase with mass. OK, if you increase the mass the Schwarzschild radius increases. So in principal it can be very large when you can see the very large object-- when you can see the very massive object.
So now-- so let us summarize. Let us summarize. So for object of mass m there are two important scales. Two important scales. So one is just the standard Compton wavelengths, and the other is the Schwarzschild radius. So one is quantum and the other is classical. The other is classical.
So let's take the ratio between them. Let's take the ratio between them. So if you take the ratio between them-- so let's forget about these two, just to consider of order up to order 1. So c squared, divided by H-bar, divided by mc. And then this again give you gm m squared divided by H-bar c. And then this is again just m squared divided by mp squared. This again is m squared divided mp squared, and p is the Planck mass.
So let's consider the different scenarios. So the first, let's consider-- just suppose the mass of the object is much, much greater than mp. OK, so in this case then the Schwarzschild radius are much larger than the Compton wavelengths. Much larger than the Compton wavelengths, And essentially, all of physics is controlled by the classical gravity, because you can no longer probe-- yeah, because a Compton wavelengths is much, much inside the Schwarzschild radius, which you cannot probe. So the physics is essentially classical gravity. And the quantum effect is not important, so you don't have to worry about the quantum effect.
I will put quote here. Not quote, I will to put some-- yeah, some quote here. You will see what this means. And the second possibility is for mass much, much smaller than p. So in this case, then the Compton wavelengths will be much, much greater than the Schwarzschild radius. OK, so this is a quantum object, so the quantum size is much larger than the Schwarzschild radius.
But we also know, precisely in this region, this lambda g is also very small. The effective strength of the gravity is also very, very small. So we also have found before that the lambda g is also very, very small. So in this case, as what we said here-- the gravity is very weak and not important. It's much, much weaker than other interactions, so you can essentially ignore them. Then we're only left with the single scale which mass is of all the mp.
And then, as we said before, the quantum gravity is important. So let me just say, quantum gravity important. If this were the full story, then life would be very boring. Even though it would be very simple. Because the only scale you need to worry about quantum gravity is essentially natural zero. It's only one scale.
And it will take us maybe hundred if not thousand years to reach that scale by whatever accelerator or other probes. So there's really no urgency to think about the quantum gravity. Because right now we are at this kind of scale. Right now we are this kind of scale. It's very, very far from this kind of scale. But the remarkable thing about black hole-- so this part of the physics is essentially controlled by Schwarzschild radius, because the Schwarzschild radius is the minimal classical radius you can achieve.
Yeah, you can probe the system, and the quantum physics is relevant. But the remarkable thing is that it turns out that this statement is not correct. This statement is not correct. Actually, quantum effect is important. So the remarkable thing is that the black hole can have quantum effect manifest at the microscopic level. Say, at length scale of order Schwarzschild radius, OK? So that's why it makes the black hole so interesting, and also makes why black hole is such a rich source of insight and information if you want to know about quantum gravity.
And as we will see, actually, we also contain a rich source information about ordinary many body systems, due to this duality. Any questions regarding this? Yes?
AUDIENCE: So when I talk about the m much larger than mp, so the m is not only an elementary particle, it can be just and object?
PROFESSOR: Yeah, it can be a bound state. So it can be-- here, we always talking about quantum object. But it can have mass very large.
AUDIENCE: That's still a quantum object?
PROFESSOR: Yeah. What you will not see from a traditional way, for such a large mass object, you will not see its quantum uncertainty, because quantum uncertainty is tiny. The Compton wavelengths is very tiny, and so the fluctuations are very small. And so you have to probe very, very small in scale to see its quantum-- from traditional point of view, we have to probe very, very small in scale to see its quantum fluctuations. And that scale is much, much smaller than the Schwarzschild radius.
AUDIENCE: OK.
PROFESSOR: Any other question? Good, so let me-- before we talk more specific about black holes, let me just make one final remark. It's that in a sense, this Planck length-- this length scale defined by Planck can be considered as a minimal localization length. OK, for the following reason.
So let's firstly imagine in non gravitational physics-- so in non gravitational physics if you want to probe some short distance scales then it's easy if you are rich enough. Then you just accelerate the particles to very high energies. Say e plus, e minus, with p and minus p. Then that can probe the distance scale, then this can probe length scales. Say of order h divided by p.
OK, so if you make the particle energy high enough you can, in principle, probe as short as any scale as you want. Anything, as far as you can make this p as large as you want. So in principle, you can take l all the way to zero. So the scale comes all the way to 0. If you take p, go to infinity.
But in gravity, this is not so. So with gravity the square of the distance [INAUDIBLE] so when your energy is much, much greater than ep-- say, the Planck mass-- then, as we discussed from there-- so this is a central mass energy, OK? Say if your central mass image becomes much, much larger than the Planck scale-- Planck mass-- then rs controlled by the image-- yeah, and [INAUDIBLE] p. Yeah, let me just forget about c. Let me just say-- OK? Then the Schwarzschild radius from now on-- so you go to y, OK?
So the Schwarzschild radius will take over as the minimal length scale. OK, so what's going to happen is that if you collided these two particles at very high energies, then at a certain point, even before these two particles meet together, they already form a black holes over the Schwarzschild sites, OK? If this energy is high enough, then we will form black hole, and then you can no longer probe inside the Schwarzschild radius of that black hole.
So that defines a new scale which you can probe, OK? So the funny thing about this Schwarzschild radius is that it's proportional to energy, rather inverse proportion to energy as the standard Compton wavelengths, OK? So after a certain point, when you go beyond the Planck mass, when you further increase in the energy, then you're actually probing the larger distance scales rather than smaller distance scales, due to the funny thing about the gravity and the funny thing about black hole.
OK, so actually, this scale increases with p-- increase with you center of mass energy. OK, and the high energies those two longer length scales. OK, this essentially defines the Planck length as a minimal scale one can probe. OK, so when your center of mass energy is smaller than the Planck scale-- than the Planck mass-- then your Compton wavelength of course is larger than lp.
But when this is greater than mp, then as we discussed here-- then the Schwarzschild radius, of course, [INAUDIBLE] object will be greater than the Planck side, and will break through the common wavelengths and will be greater than the Planck sides. And this give you, essentially, the minimal radius to probe. Alternatively, we can also just reach the same argument. Simply, I can just write down a couple equations.
So let's consider you have uncertainty. So suppose you have a position, data x, and then the answer in the energy or momentum associated with the data x is data p. But on the other hand, the distance you can probe must be greater than the Schwarzschild radius associated with lp, data p. OK, data p. So if you combine these two equations together-- so this is greater than GN H-bar divided by lp. So now I have suppressed the c.
So you can see from this equation-- you can see that data x must be greater than H-bar GN, which is lp. OK, which is lp here. This is the same argument as this one, but this is a little bit formal. So the essence is that once you're energy is big enough, then you will create the black hole, and then your physics will completely change.
AUDIENCE: [INAUDIBLE] black hole evaporates [INAUDIBLE] they do not contain that information.
PROFESSOR: Right, yeah so we will go into that. When the black hole evaporate, we still don't probe the short-- it's still harder to probe the short distance scale. Yeah, we will talk about that later. So yeah, here just a heuristic argument to tell you that because of this, actually the physics are very special. The physics of the gravity is very special. Any another questions?
AUDIENCE: As a probe for quantum gravity, I was thinking-- what if there were some phenomenon in which gravity is weak, and it's a macroscopic scale, but there's some kind of coherence happening that will-- maybe on the scale of galaxies or something like that-- that will allow quantum effects to manifest. Like an analogy of what happens in a laser or something like that.
PROFESSOR: Yeah, that is black hole. The black hole is the way which gravity can manifest at the quantum effect that matches in scales. And we don't know any other ways for gravity to manifest such quantum physics at large distance scales. OK, so let me conclude this I generally discussion. Again, by reminding you various regimes of gravity. Various regimes of the gravity or quantum gravity.
So in this discussion, you should always-- in the discussion I'm going to do in the next minutes, you should always think, when I take the limit I always keep my energy fixed. I keep the energy scale I'm interested in fixed. I keep that fixed. So the classical gravity regime is the regime in which you take H-bar equals zero-- take Newton constant finite-- and the regime of a particle physics, we would normally be, sometimes, say, QFT in fixed space time.
So this is a quantum field theory in the fixed space time, including curved-- including curved. So this is a regime in which H-bar is finite, while you take the Newton constant go to 0. And then there's, of course, the quantum gravity regime, which is the GN and the H-bar both finite. And then there's a very interesting regime, which is actually the regime most of us work with.
So there's also something called the semiclassical regime for quantum gravity. So this is the regime you keep H-bar finite, and you expand this system in Newton constant, OK? So around-- so you expanded whatever quantity Newton constant around-- say G Newton equal to zero. So G Newton equal to 0 is the classical regime. It's the regime the gravity's not important, but including H-bar.
And now you can take into account the quantum gravity fact, semiclassically, by expanding around the GN. OK, so this is normally what we call semiclassical regime of gravity. Yes?
AUDIENCE: Do we know that G goes to 0 [INAUDIBLE] and it's not some other part?
PROFESSOR: Yeah, this is a very good question. So this is indeed the question. Indeed, most of the particles regarding black hole is in this regime. So our current understanding of quantum physics or black hole is in the semiclassical regime, and you treat any matter field H-bar finite, but the gravity's weak. And so there are various indication that this limit is actually not smooth, but the only for very subtle questions.
For simple questions, for typical questions, actually this is a limit. This limit is smooth, but there can be very subtle questions which this limit is not smooth. And one such question is this so-called the black hole information loss, and the subtle limit of taking this-- yeah, you [INAUDIBLE] taking this limit. Any other questions? And this is a regime, actually, we will work with most. OK, this is a regime we will work with most. So we will always-- in particular in nature.
So right there I'm keeping the H-bar explicitly, but later I will also set the H-bar equal to 1. And so H-bar will equal to-- so we are always typically working with this regime. So H-bar equal to one. And then you will have-- yeah, then you take into account the fact of GN in the perturbations series.
Good, so now let's move to the black hole. OK, with this-- yes?
AUDIENCE: [INAUDIBLE] Also working in the other sermiclassical regime. I mean finite GN, but expand H-bar.
PROFESSOR: Yeah, this is not so much. It's because it's easy-- in some sense it's easy. So in a sense, we are doing a little bit both. Yeah, so later-- right now I don't want to go into that. You will see the effective coupling constant and show the quantum gravity fact is in fact H-bar times GN. It's H-bar times GN. And so the quantum gravity fact will be important when the H-bar times-- when you do perturbation series H-bar times GN.
So when I say you are doing same thing GN, essentially it's because I'm fixing H-bar. So you're actually doing perturbation series H-bar GN. So you have to do both. Any other questions? Good. Right, so now let's talk about the black hole. Let's talk about classical geometry. OK, so here I will assume you already have some background in GR-- in general relativity-- and for example, you have seen since Schwarzschild metric, et cetera.
And if you have not seen a Schwarzschild metric, it's also OK. I think you should be able to follow what I'm going to say. So for simplicity, right now let me consider zero cosmological constant. OK, zero cosmological constant. OK, zero cosmological constant corresponding to-- we consider symptotically Minkowski space time. And so in the space time of zero cosmological constant entered in the space time metric due to an object of mass m. It can be written as-- so this is the famous Schwarzschild metric.
And so of course here we are assuming this is a spherical symmetric, and a neutral, et cetera. So this object does not carry any charge. And this m and this f-- it's given by 1 minus 2m, mass divided by r, or it's equal to rs divided by r. OK, so this rs is the Schwarzschild radius.
So now c is always equal to 1. So if this object-- so we consider this object is very close metric center. If this object have finite sides, then of course this metric only is varied outside this object. But if the sides of this object is smaller than the Schwarzschild radius, then this is a black hole.
OK, and the black hold is distinguished by event horizon and r equal to rs. OK, and the r equal to rs. So at r equal to rs, you see that this f becomes 0. OK, so f equals become 0. So essentially at here gtt-- so the metric for the tt component is becomes 0, and the grr, the metric before the r component become infinite. And another thing is that when r becomes smaller than rs, the f switch sign. So f become active, and then in this case then the r become time coordinates and the t becomes spatial coordinates, when you go inside to the r equal to rs. OK, this is just a feature of this metric.
So now let me say some simple fact about this metric. So most of them I expect you know-- I expect you know of them. But just to remind you some of those facts will be important later. OK, so these are mostly reminders. So first, this metric-- if you look at this metric itself, it's time reversal invariant. OK, because if you take t go to minus t, of course the invariant on the t goes to minus t.
OK, so this-- actually, because of this, this cannot describe a real black hole. So the real life black hole arise from the gravitational collapse, and the gravitational collapse cannot be a time symmetric process, OK? So this cannot describe-- so this does not describe a real black hole, but it's a good approximation to the real life black hole after this object have stabilized. So after the gravitational collapse has finished. So this is a mathematical-- in some sense, it is a mathematical idealization of real life black hole. OK, so this is first remark.
So the second remark is that despite this grr goes infinity, this metric component goes infinity. So the space time is non-singular at the horizon. OK, so you can check it by computing, say, curvature invariants of this metric. You find the number of the-- all the curvature invariant that are well-defined. So this horizon is just the coordinate-- you can show that this horizon is just a coordinate singularity. Which we will see-- actually, we will see it in maybe next lecture, or maybe at the end of today's lecture, that just this coordinate, r and the t, coordinate become singular. The coordinate itself become singular.
So this t, we normally call it Schwarzschild time. So let me just introduce a name. So this t, we call it the Schwarzschild time. So Schwarzschild found this solution while fighting first World War, really in the battlefield. And a couple months after he finished this metric, he died from some disease. Right, this is that. So another thing, you can easily check yourself with r equal to rs-- the horizon is a null surface-- is a null hyper surface. It's a null hyper surface.
The null hyper surface just say this surface contains geodesics which are null. And this is a-- the third remark is an extremely important one, which we will use many times in the future. We said the horizon is a surface of infinite red shift compared to the-- infinite red shift from the perspective of observed infinity, OK? So let me save time, now, to add to this qualifying remark from the perspective of observed infinity.
So now let me just illustrate this point a little bit more explicitly. So let's consider observer-- consider an observer-- so let me call oh-- at some hyper surface r, which is close to the horizon. Yeah, say as someplace which is close to the horizon. Slightly outside the horizon. And let us consider another observer, which I call o infinity, which at i go to infinity. OK, very far away from the black hole.
So let's first look at this observer. So the i equals infinity, then your metric-- so at i equals infinity, this f just becomes one, because when your r goes to infinity, this r just become 1. And then this become the standard Minkowski space time, written in the spherical coordinates. So we just have the standard Minkowski time written in spherical coordinates.
And then from here you can immediately see-- so this t is what we call Schwarzschild time. t is the proper time for this observed infinity. Say, for o infinity. OK, so now let's look at someplace i equal to rh. So at i equal to rh, then the metric is given by minus f rh dt squared with the rest. OK, and to define the proper time for the observer at-- we can just directly write it as minus d tau squared. So that's the proper time observed by observer at this hyper surface, OK?
So then we concludes-- so let me call it tau h. So we conclude that the problem time for oh is given by f 1/2 rh times dt. OK, so it relates to the proper time at infinity by this factor. So if I write it more explicitly-- so this is 1 minus rh, divided by rs 1/2 times dt. So we see that as rh-- suppose this observer-- the location of this observer approach the horizon, say, if rh approach to rs, then this d tau h divided by dt will go to 0.
So that means compared to the time at infinity the time at r equal to rs becomes infinite now. So-- or approximate, let me say, becomes infinite now. So that means any finite interval-- any finite proper time interval for observer at oh-- for this oh-- when you view [INAUDIBLE] infinity become infinitely null, OK? Become very long time scale. OK, become very long time scale.
So you can also invert this relation. OK, you can also invert the recent relation. Say, suppose some event of energy with energy eh-- with proper energy eh-- say, for this observer at oh, for this observer oh. Then because of the time relation between them, because of the time relation between them, then from the perspective of this observer at infinity the energy is given just but you invert the [INAUDIBLE] between the time, because of the energy and time conjugate.
So the e infinity becomes eh times this f 1/2 rh. So for this just again says, in a slightly different way, that for any finite eh local proper energy-- so this is a local proper energy for the observer at oh-- this e infinity-- the same event viewed form the perspective of the observer at infinity goes to 0. S rh goes to rs. So that e is infinity red shifted-- become infinite red shift.
So any process with local proper energy viewed from infinity corresponding to very, very low energy process. So this actually will play a very important role. This feature will play a very important role when later we talk about holographic duality and it's implication, say, for the field series, et cetera. Yes?
AUDIENCE: So this is just a pedantic comment, but I think you need a minus sign in front of your f, just to make sure proper time isn't imaginary.
PROFESSOR: Sorry, which minus sign?
AUDIENCE: In the d tau stage.
PROFESSOR: You mean here? You mean here or here?
AUDIENCE: Below that.
PROFESSOR: Below that, yeah-- oh, sorry, sorry. Thank you, I wrote it wrong. It should be rs rh. Yeah, because rs is above. Sorry, yeah-- so rs is above, so varied rh, so rh is downstairs. Thank you, so rh is-- so I always consider rh as [INAUDIBLE] equal to rs, OK? Any other questions? OK, and so some other fact. And again, I will just list them. I will just them.
If you're not familiar with them, it should be very easy for you to go through them, to re-derive them, with a little bit knowledge in gr. So number four is that it takes a free fall-- free fall means we just follow geodesics-- free fall of a traveler a finite proper time to reach the horizon-- say, from the infinity-- but infinite Schwarzschild time. So also you can easily check that it actually takes infinite Schwarzschild time for object to fall through the horizon. But from the free fall observer itself, it's actually just finite proper time.
And so from the perspective of the observer at infinity, it looks like this object never fall into the black hole. It's just frozen at the horizon. So another remark is that once inside the horizon-- that means when r becomes smaller than rs-- the traveler can not send signals to outside, nor can he escape. So that's why this is called even horizon.
So we will see this slightly later. In next lecture we will see this explicitly. So finally, there are two important geometric quantities associated with the horizon. Two important geometric-- OK? So the first one is the area of the spatial section. So suppose-- so let's consider we are at i equal to rs, and then here you just said r equal to rs, and then this is a two-dimensional sphere, OK? So this two-dimensional sphere corresponding to a spatial section of the horizon.
So first, A is the area of a spatial section. So you can just say let's look at the area of this part with the r equal to the horizon radius. So this just give you a equal to ah ah, equal to 4 pi rs squared. And this rs is 2GN, so this become 16 pi GN squared. OK, so this is one of the key quantities of a black hole horizon. It's what we call the horizon area, OK? What we call this horizon area.
And the B is called the surface gravity. B is called service gravity. So the surface gravity is defined by the acceleration of a stationary observer at the horizon as measured at infinity. OK, at infinity means at spatial infinity. So you can-- if you are not familiar with this concept of surface gravity, you can find it in standard textbooks. For example, the Wald say page 158, and also section-- OK. Just try to check it there.
So, say, suppose you have a black hole. Say this is the Schwarzschild radius. Of course, things want to fall into the black hole. So if you want to remain at a fixed location outside the black hole, then you really have to accelerate. You have to fire some engine to keep yourself to stay there. And you can calculate what is acceleration you need to be able to stay here, OK? And once you are closer and closer to the horizon, that acceleration becomes bigger and bigger-- eventually, becomes infinity when you approach the horizon.
But because of this red shift effect, when this acceleration is viewed from the units for observer at affinity, then you have infinity divided by infinity, then turns out to be finite. And this is called a surface gravity. It's normally called a kappa. Normally called a kappa. And this is one of basic quantities-- basic geometric quantities of the horizon. So I will not derive it here. I don't have time. And if you want to see this, Wald's book.
So you can calculate that this is just given by 1/2 the derivative of this function f evaluated at the horizon location. OK, so f is equal to 0 at the horizon, but f prime is not. OK, so you can easily calculate. So this is 1 over 2rs from here, and is equal to 1 over 4GN. OK, so this is another very important quantity for the black hole. Actually, I think right now is a good place to stop. OK, so let's stop here for today, and the next time we will describe-- then from here we will discuss the causal structure of the black hole, and then you will see explicitly some of this statement, if you have not seen them before.
Free Downloads
Video
- iTunes U (MP4 - 175MB)
- Internet Archive (MP4 - 175MB)
Subtitle
- English - US (SRT)