Flash and JavaScript are required for this feature.
Download the video from iTunes U or the Internet Archive.
Description: Module manufacturing: encapsulation materials, availability, trends. Systems: Grid-tied and stand-alone, tracking and non-tracking. System components, including balance of systems components. Design criteria, tradeoffs, costs. Building integration, BIPV. Scaling, and power grid integration. Appropriate technology selection. Life cycle analysis. Failure: failure modes in stationary and tracking systems, accelerated testing, field testing, service and warranty contracts. Fire safety.
Instructor: Prof. Tonio Buonassisi
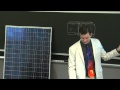
2011 Lecture 17: Modules, S...
The following content is provided under a Creative Commons license. Your support will help MIT OpenCourseWare continue to offer high quality educational resources for free. To make a donation or view additional materials from hundreds of MIT courses, visit MIT OpenCourseWare at ocw.mit.edu.
PROFESSOR: So modules, systems, and reliability. What we're going to do is talk about how we go from the cells, or from the films, to full modules and, finally, to systems. So our first learning objective is to describe, more or less, the DNA or anatomy of a PV module. This will be a bit of a prep for the visit to Fraunhofer CSE, where we'll get to see the labs and see all the materials that go into a PV module. So just establishing some definitions up front so we're all speaking the same language-- you start out with either a film. Right? If you're depositing a thin film material, which is usually divided into discrete devices using laser processing, or you can start out with a discrete wafer which has been processed into a cell.
The module is the combination of many of these cells, shown very generically right here without the interconnects. Because you can tie up the cells together in parallel or in series, depending on what sort of voltage and current outputs you desire from your module. And then finally the modules are situated together in an array. That's showing a combination of six modules forming that array right there.
And as you can see, modules are typically this size right here. They're usually about this size or even a little larger. They're rather heavy, so you can get a sense. They're not extremely light. The majority of that mass is coming from the glass in the front, some from the aluminum as well.
And they're comprised of many materials. If you were to take this apart-- which we'll actually see the individual components at Fraunhofer CSE-- you'll notice that there are many materials involved in making one of these modules. From the back skin materials, the junction box, the aluminum framing on the side, the glass on the front, the encapsulant materials that fuse everything together-- that bind everything-- and the cells, the solar cell devices themselves.
So our first step is to walk through the anatomy of a module, at least in theory, so that when we actually see it at Fraunhofer CSE, we'll have a better sense of what we're looking at that. Yeah. Ashley?
AUDIENCE: Here, what determines that specific size? We've already talked about the pseudo-square and how you have to balance those two different cost parameters. But why that size specifically?
PROFESSOR: Why that size specifically? We're going to get to that in a few slides. It comes, as you probably guessed, from some historical reasons.
AUDIENCE: OK.
PROFESSOR: So some basic principles about solar modules-- there's quite a diversity of modules when you look at them. These are examples just within one company, just to highlight the range of module types that you might see. Right here, you can see in this particular module, there's very little anti-reflective coating on the glass. So you're able to look through the front glass and see some white spaces in between the cells.
We know, from the lecture on crystalline silicon PV, that when you see these little gaps in between the cells, those are pseudo-squares. Right? Those are those round ingots of Czochralski silicon that have been sliced into wafers. And then the edges have been shaven off, and you have that stop sign type pattern of a solar cell. Right? So that way you know, OK, these are single crystalline cells lined up in a module together. And there's very little anti-reflective coating on the glass, which means that you're losing a fair amount due to reflectance.
The module on the right-hand side right here has that anti-reflection coating on the glass. It could be an index of refraction gradient to absorb more of the light. It could also be surface texturing to scatter the light and trap it by total internal reflection once it gets inside. There's a few different ways that one can do that. Usually with a film coating is the most common.
And here on the left-hand side, we have a similar sub-cell component but much larger module comprised of a larger number of cells with a higher power output. And we can see quite a wide variance of different types of modules. That will be an important message in a few.
The water rinse cycle really depends on where you're at. For the modules on my roof, we just leave them up. Here in New England, it's pretty much OK. If we did have trees that deposited leaves nearby, we'd have to clean it off in the fall, but we don't.
If you're mounting modules in the Middle East, where that fine-grained sand exists and, if you're within 10 kilometers from the coast where you have the salt and the seawater in the air, you can get this really hard-to-remove sand caking the modules. And you would have to clean them much more frequently than this. As a matter of fact, I believe the numbers are-- in Abu Dhabi, the capital of one of the Emirates in the UAE, if you were to just leave your modules out near the ocean, near the desert, you would have a 40% drop in module output over a month.
So that's what happens in certain environments. In certain others, like New England, we're more buffered in that regard. We have frequent rain, and we don't have the dust coming from any nearby desert. Typically, there are no moving parts, and typically there's a 20- to 30-year manufacturer warranty. Some of the newer materials that have been less tested might give, say, a 10-year manufacturer's warranty and have to offset the risk in years 10 to 20 by lowering the cost up front or lowering the price up front.
So we have some basics about solar modules just to situate ourselves. Let's dive into the module DNA, since this is where the rubber hits the road. I'm going to show this to you in theory, and then we're going to see reams of these module innard materials when we visit Fraunhofer.
So we start with the solar cells themselves. These are these little blue objects right there. Typically, these cells are already strung together at that point. They have contact metallization on the front side, which we deposit, say, for example, by screen printing, which you've done in the lab downstairs. And then you string them together using a machine called a tabber-stringer to connect one cell to the next, essentially the front of one cell to the back of the next. And they're all lined up like that.
And you deposit layers of EVA, which is ethyl vinyl acetate. This is typically the encapsulant material used. It's shown in red right here in this drawing. It's the encapsulant material used for crystalline silicon PV.
On the back side, there is a sheet of material called Tedlar. Despite it being drawn in yellow right there, it's the white skin material here. It's this white material right around here. And we have the glass on the front side. And typically, it's low-iron glass so it can transmit the ultraviolet light, or a large portion of it, to give better blue response to your solar cells. So you're capturing a larger portion of the solar spectrum.
OK. So we have the different components right there. One little piece of trivia about EVA-- for the chemists in the room, what do you think this would decompose into? Let me just give you a little hand here. If you had some-- let's see. All right. So these represent methyl groups, hydrogens, carbon, carbon, carbon, double bonded to an oxygen. Anybody recognize?
AUDIENCE: It looks like acetone?
PROFESSOR: Yeah. So if you're looking at the decomposition of this little group right here, you could easily envision it decomposing into acetic acid from the name ethyl vinyl acetate. So if you have a thin film material that could react with the encapsulant, you could decompose your encapsulant and cause a degradation of not only the encapsulant itself, which would block some of the light going through, but of the solar cell absorber material, too.
So that's why, in many thin film materials, there are other encapsulants used, like PVB, polyvinyl butyral. And some folks are even talking about getting away from glass and EVA altogether and just putting down, say, an organic resin-type material. Maybe a very hard polymeric material that could be used in a floor in a clean room, let's say. Yeah.
AUDIENCE: Is the low iron content of the glass also important to prevent all impurities getting into the silicon or are we past that point?
PROFESSOR: So the question was is the low iron content in the glass also useful for preventing impurities from getting in. I would say, if you're producing-- the diffusivity and solubility of impurities in a solid typically follow Boltzmann statistics, meaning they increase exponentially with temperature. It's an entropy-driven effect.
And as a result of being at room temperature, the transmission, or the diffusivity and, hence, the solubility of iron inside of the bulk silicon is very low. And, even over a 20-year span, the total diffusion length of the iron from the glass into the silicon would be rather small. Now, point taken, if you were to, say, create a quartz tube to do your phosphorus diffusion at 800 degrees C or 850 degrees C and you had iron inside of that quartz tube, then it could very easily diffuse into your wafer. So it is important to be considerate of impurities and the effects thereof but at low temperatures-- close to room temperature-- there's very little risk of it moving around. Good.
So Tedlar, this back skin material right here, forms an almost impenetrable back layer. You might want to put little asterisks around-- sorry, little quotes around impenetrable. Nothing is absolutely impenetrable but it is a very tough, tough material. If you were to take a piece of Tedlar and try to break it, try to rip it or tear it, even the strongest in the class would be challenged in that regard. And we'll have a chance to do that at Fraunhofer.
The aluminum frame provides rigidity. Right? So the mechanical engineers in the room would understand that the bending mode of this glass, or the twist, is prevented by these rigid extruded aluminum components right here in the back. And you can see that a lot of the mass is concentrated away from the centroid, which results in a larger stiffness, prevention of a larger bending moment-- second moment, if you will. OK.
So there's a fair amount of mechanical engineering that goes into the design because you want to minimize commodity material costs. You want to minimize the amount of materials that go into the module, but you still want it to last for 20 years. You still want it to be resistant to snow loads, to wind loads. You don't want it to break, and you don't want to make good on your 20-year warranty. That costs you money as a manufacturer.
So the circuit design in most modules follows something very similar to this. Now, what you see when you glance at this, what you should be looking at is-- here are the contacts. Those are the leads coming out of the module. They're essentially these leads coming out in the back of the junction box. If you want to turn it around again, here we go.
So here are the module leads coming out. And the question is, how do I string together the cells inside of my module? How do I string together these cells right in here to provide the greatest value to the customer?
Do I connect them all in series and make just one long, snaky electronic path? Do I connect them all in parallel? But that would require a lot of wiring. Do I do something in between?
And if you were to come up close to here and inspect it, what you would see is that you have strings in parallel. So you have one going from here, down and around. That's one, two, and three. Right? Three of these strings, and they're connected-- those three strings are connected in parallel. Each string is comprised of several solar cells.
Now, for historical reasons, the crystalline silicon solar cell modules have typically had strings of around 36 cells connected in series. What this does is it yields a maximum power point voltage somewhere in the range of 17 to 18 volts. If you remember the VOCs of your solar cells and that maximum power point, the voltage of your solar cells are some around 0.5.
And so you take 36 cells, divide by 2-- or multiply by 0.5, if you will. And you're landing somewhere in this ballpark. And what that does is it enables you to hit, even under lower light conditions, the overpotential needed to charge a rechargeable battery.
So if there's a 12-volt battery and the voltage output of the battery is between, say, 12 and kind of dropping down to 10 before it starts using its utility-- when you try to start your car and it doesn't really turn over and you can start your engine, it's probably because the voltage in your battery has dropped to about 10 volts. The overpotential needed to charge it, 13-plus, that's what you're hitting with the string. And that's, for historical reasons, why we had this number of cells connected together.
As grid-tied systems become more common, though, this constraint is being reduced. Over 90% of all of our solar modules today are connected to the grid, including the ones in my home. So when I'm not there and it's producing excess electricity, it's pumping it into the grid. And maybe we're using some of my electrons here. I don't know.
So as grid-tied systems become more common, you care less about meeting the overpotential requirements for a battery. You care more about matching the output of your solar module with whatever inverter technology you're using to convert the DC power into AC power.
Number two, why do they spec the modules that size? You notice that I could lift it, just barely. I'm a university professor. I sit in my chair and think most of the time. A person, somebody strong out there in the field mounting modules, say if Jessica in the back were a module installer, she could lift this no problem probably with her pinky finger.
And that's why they're bite-sized, right? They're a single-person, liftable, installable modules. You can't make them too big, or else you start needing cranes which, of course, has its own benefits for large field installations. Wouldn't really make sense with our current mode of installation on residential.
AUDIENCE: [INAUDIBLE]
PROFESSOR: Yeah.
AUDIENCE: If you had a battery-- if you were charging a battery with the module, you also wouldn't want to have too many volts, right? Maybe that's a really basic electronics question.
PROFESSOR: Yeah, so the question is, do you benefit by having larger overpotential when recharging a battery? Well, I'm not a battery expert, so I'll definitely caution on this. I would imagine that there would be some law of diminishing returns. You would be limited in terms of the recharge rate, not by the overpotential but by some other component like the electrolyte diffusivity, let's say, inside of your system-- as an example. But I would defer that to the battery experts. My impression, though, is that you get a law of diminishing returns.
Packing fraction in the modules. So we talked about the different cell sizes if you're processing discrete cells. The higher packing fraction enables lower glass encapsulant and cost per watt peak.
The lower packing fraction, you get to play some games with optical concentration. For here, for instance, if you have space between your cells, you can have a diffuse Lambertian scatterer in the back which will reflect the light at a different angle, scattering it. And that light could be trapped by total internal reflection inside of the glass if the angle reaching the glass is, say, 15 degrees or greater. So you can play some games there. That's why the Tedlar back skin being white is typically used in most solar modules, so that we reflect and then scatter and then trap the light that comes in in between the cells.
So to put this into another fancier, three-dimensional framework-- we have our interconnected cells. We have our encapsulant above and below. The encapsulant material is an interesting thing. It's solid at room temperature but it begins to flow at around 150, 175 degrees C which means, if you've ever put a plastic bottle in the oven and you've noticed how it begins to melt and sag and droop, that's essentially the type of flow that you would experience inside of this encapsulant material.
And it flows around the cells. And it fuses them together with the glass on top, and they stick together once they cool down to room temperature. That forms what's called a laminate. Because now you have the back skin-- the frame isn't shown right here-- you have the back skin material. You have the encapsulant, the cells, the encapsulant on the other side, and your glass on the front side all together forming a nice stack of materials. And they're stuck together, or bound, by the encapsulant. That's called a laminate.
It doesn't resist bending very well, or twist very well, because it doesn't yet have the frame on it. But it certainly can be transported around the factory and moved from one station to another without risk of, say, the cells falling out. They're pretty well fused there.
In terms of moving to new materials, there's a lot of work right now to squeeze the commodity costs out of solar module manufacturing. If you look at the percentage of cost of commodity materials in the solar module, it is quite high. And so people are focusing on new types of transparent front surface materials that let the light in, glass replacements. Right? You could envision-- we have many examples of really hard, resistant, polymeric materials in our daily lives. People are looking into those.
The encapsulant materials-- ethyl vinyl acetate is fairly expensive, and there aren't that many companies that manufacture it. And so people are looking to options to replace it. Tedlar even more so, that back skin material. Although the challenge there is a lot harder, a lot tougher. No pun intended.
It's a really strong material. And a lot of our modern chemistry or chemical engineering goes into making and designing it. And then finally finding some replacement for the frame. People are talking about frameless modules. That means taking off this aluminum piece right here on the side and just leaving the laminate and somehow providing the mechanical rigidity to the laminate and avoiding the cost of the extruded aluminum components. So a lot of work going in in module design.
This is a picture of folks mounting the aluminum framing onto the module. These are essentially large steel components that are pushing the aluminum into the module and making sure that there's a snug fit between the aluminum and the module itself. As you can see by the size and shapes of these cells, that gives you an indication of what company that is.
Module technology-- there's some work as well going into replacing-- let's see, here we go-- replacing the tabbing-stringing step. Right? So this is a machine the tabs and strings the cells to one another. We'll see one at Fraunhofer. It's really impressive when you see it go, but it's also quite expensive.
And so if you could get rid of the tabbing stringing-- imagine just lining up the little cells like cookies on a cookie sheet. And they all are interconnected by contact with whatever back skin material is on the back that is printed, let's say, with the circuitry already. You would wind up in a situation like this, where you have your cells in direct contact with some printed circuitry on your back. Boom, boom, boom, boom, boom-- and you're off and running.
In this particular case, you see these little white dots here. The grid pattern on that cell is a radial spiderweb pattern that emanates from that central point, collects current from nearby-- essentially, an area about that big is collected, let's say by that little point right there. And then there's a hole drilled through the solar cell where the metal is wrapping through to the back. And that's where it's making contact with the back side right there.
And there are other regions of the solar cell that are making contact with the bulk, the base, if you will. So you have both of your n- and your p-type contacts on the back side of your solar cell. And that's what's shown in this drawing. So there are attempts, as well, to put all the contacts, or connect the contacts, onto the back to enable this monolithic manufacturing. Yes.
AUDIENCE: How does that not short?
PROFESSOR: OK. So how is that not a short? Let me show you how that would not be a short. What I'm going to do is attempt to draw two different cell designs that could lead to not shorting.
One cell design that could lead to not shorting would be-- let's say this would be our front surface. Our base is-- I'm going to draw it flat here in the back just for simplicity, but it could be textured as well. I'm going to call this a lightly doped semiconductor material.
And then I could have heavily doped-- let's say this would be-- I'm going to exaggerate here just for effect. This could be my heavily n-type doped material. This could be my heavily p-type doped material. So I'm drawing p plus, n plus, another n plus, another p plus.
So I have charge separation occurring at these regions right here and then contacts on the other side. This is my electron contact. This is my hole contact. This is my electron contact. This is my hole contact.
And now what's happening is photoexcited carriers are being generated in here. But the field that separates them is all in the back. And so now the carriers are being separated, electrons being collected by the darker-shaded, rather, the shaded areas, and the holes being collected by these unshaded contacts here in the back. And, if I'm clever about my contact positioning, here I have kind of an interdigitated electron and hole collectors. Right? I'm able to collect both without having any sort of shunting. Another design might be-- let's see. So this is called an Interdigitated Back Contact structure, or IBC.
AUDIENCE: What is it, [INAUDIBLE]?
PROFESSOR: Actually, less relevant in this particular case. In most interdigitated back contact cells, it's actually n-type silicon, lightly doped n-type silicon, just because point defects tend to have smaller capture cross sections than n-type silicon and p-type silicon. So minority carrier lifetime tends to be larger.
Another cell design, again, looking at cross section, you might have-- this being your solar cell-- you could add texture, but I'm omitting it just for simplicity. Now, let's see, we drill a hole using a laser right here. So we have a very thin hole.
Of course, in three dimensions, there's mechanical rigidity by the bulk of the material in the other dimension. But we have this hole drilled through-- and we can make about 18,000 of these holes in about six seconds with modern laser technology, so it's really not that hard to make this happen. Although a lot of engineering effort is put into it, but it is possible. And then you could have your contact metallization, say, for electrons right here.
You could have the p-n junction like this. So this, here, could be your n plus material. Again, n plus material, this, by effect, would also be. And you can have your p materials over here. And you could make contact to the back right here and contact to the back right here.
And as long as there's no metal short between the electron and hole-- say, for example, you generate an electron hole pair here, the electron goes up to here. It goes through the metal to the back and gets pulled out. And the hole goes to this contact over here, and that's how you separate the charges. So this would be an example of an emitter wrap through or a metal wrap through device technology. Also shown-- MWT, Metal Wrap Through.
So we have at least two different device geometries and many more device architectures that could fit in with this future vision. The problem right now is that the printed back skin materials cost about five times more than the non-printed ones just because they're very low scale. People haven't investigated it much. So any time you introduce a new technology into the market-- you'll hear it over and over again in these innovation and entrepreneurship classes here at MIT-- you have to be significantly better than the competition.
If you're only a little bit better, you face an enormous barrier to entry because you have the rest of the industry pedaling along on their bicycles in a huge peloton, benefiting from each other's drafting, and you're on your own bike facing your own headwinds. Right? Trying to build everything up-- your own factory, your own expertise. It's challenging.
But I'm not trying to put a damper on innovation. I'm just trying to qualify the areas of greatest opportunity and lowest resistance to change. Go ahead, prove me wrong. Make a difference.
Without that sort of headstrong mentality of, gosh, I know I have something here and I'm just going to prove the world that I have it, we wouldn't have, for example, the Pilkington plate glass process working-- or float glass process, sorry. We'd still probably be pouring and grinding and polishing our panes of glass and they'd be very expensive.
That was a little side story, but it took them about 10 years to develop the float glass process inside of a company owned by a family, as opposed to a publicly traded company. So they could continue losing money over a long period of time until they perfected it. And once they got it right, the whole world copied it, but it took 10 years of good investment for them to get it right. All right. Let's move on.
We're going to talk about module spec sheets here. When you came into class today, you should have picked up one of these. This is an example of a First Solar module spec sheet. What it does is it hits you front and center. The company name-- First Solar. FS Series 3-- that's the name of the module, and PV module.
So it gives you the mechanical description, the size, the weight. And then, on the back, it gives you all of the technical parameters that you've wanted. So on the backside of the-- we'll go to the First Solar spec sheet.
OK. Well, on your spec sheet right here, you can look at some of the parameters. There's power at the maximum power point, voltage and current at the maximum power point, the VOC, the ISC, and so forth. You have a bunch of different parameters including the temperature sensitivity. Right? How much does the power degrade with increasing temperature?
So all of these things we've studied in class, and now when you pick up the spec sheet you're a pro. You look at it, and you're like, oh yeah. I know what that does. I know what that means. I know how to model the energy output of the system.
And that's essentially what the engineers do when they pick up the spec sheet. They read it. And then they input those parameters into their computer models, and they can predict how these modules will perform out in the field at a given location.
OK. And so you have a variety of different spec sheets. These are the modules that are on my roof. They're Sharp 187-watt modules. You have, for example, right here an example of an Evergreen Solar module.
Let's spend a minute here looking a little more deeply. These modules, these Evergreen Solar modules, are the same size, but there are three different power outputs. And I think we went through this already.
We have good cells, medium cells and bad cells. And they're binned together in the different modules so that they can extract the maximum price from the consumer. So that's why you have the three different ratings of power for three different types of modules with, essentially, the same form factor, the same size and weight. They're just different-quality cells. Yeah. So that's that. Temperature coefficients, so forth.
OK. One thing to note right here is, on the back sides of your modules, you typically have a little diagram that shows you how to interconnect one module to the next. And those are the cables that are used to do the interconnection. There are some companies trying to get fancy right now and saying, well, instead of interconnecting and then affixing the modules separately, why don't we just make these modules that kind of click together?
And part of the clicking process is the interconnection electrically, and the other part, obviously, is the mechanical rigidity. So there's certainly room for optimization on module design. Yeah. Jessica.
AUDIENCE: When they're sold-- when modules are sold, they're advertised for their voltage at maximum power point, right?
PROFESSOR: Mm-hmm.
AUDIENCE: What's a software that we should use-- I'm actually using this for another class. What's the software that we should use that maximum power point voltage into-- translate that into what it's actually going to be? [INAUDIBLE]
PROFESSOR: OK. So the question became how do I go from a module spec sheet, like this, with this data right here, and translate that into number of kilowatt hours produced per unit time-- day, year-- at a given site location. The best program that I know of that encompasses all of this would be a program called PVWatts, produced by NREL. PVWatts, all together, and you can look it up, you can use it online, and you can input your specific module spec parameters into the program.
Now, that said, we have a lot of the tools to do the estimates ourselves. If you ruffle back to homework-- I think it was homework number two, we calculated the output of a solar system based on the module spec parameters. And so now that we can pick up a module spec sheet and read it in a bit more detail, we can perform those estimates a little bit more accurately.
Yep. Omar.
AUDIENCE: Your first solar panel is a lot smaller than that panel right there?
PROFESSOR: Yep.
AUDIENCE: So is there a reason why First Solar goes with smaller cells?
PROFESSOR: Yeah. So why is this First Solar module, the FS Series 3 module, a little smaller than some of the crystalline silicon modules? For thin film deposition, especially for this closed-space sublimation process that First Solar uses, you're limited in terms of the deposition area by the uniformity of deposition over a large region. So if you're sputtering the material, it's typically related to the size of your target and the distance to your substrate. That will limit the maximum size that you can deposit uniformly.
And you probably want to deposit within plus or minus 2%, let's say. Maybe 5%. I'm not extremely privy to the precise tolerances in the PV industry in terms of thin film thickness variation, but that would be my estimate. And in terms of these thermal evaporation processes, again, it's how the machine is designed. So I imagine you could probably go bigger. Is it cost effective? Question mark.
Somebody at First Solar most obviously did the cost analysis and said that this is the optimum between a variety of different factors, between manufacturability and ease of installation. Yeah.
AUDIENCE: Is there any reason why they don't put efficiency on this sheet?
PROFESSOR: Is there a reason why they don't put efficiency on the sheet? Well, you could probably very easily calculate the efficiency, right? There are two types of module efficiencies. There is what's called total area efficiency, where you take the total area of your module all the way up to the aluminum frame and calculate that as your area. So you take your power-- your maximum power rated at AM 1.5 sun conditions and divide it by the area.
There's also a variety of other less straightforward ways of calculating efficiency, some of which take the active area of the cells only. Others exclude the frame. And so the module efficiency is not extremely straightforward parameter. It has to be specified based on what areas is assumed.
That would be my estimate for why they didn't put it right on here. And secondly, their module efficiency, if you do the math, that one probably turns out to be somewhere between 11% and 11.5%. Now they're hitting 12% or so. That's not a very high number compared to, say, a crystalline silicon module. And so it wouldn't be probably something they advertise in their spec sheet.
Describe how PV module power output is affected by the cell mismatch losses. Warning-- I'm going to lose some of you on this. Those of you without a very strong electrical engineering or physics background, I'm going to pick you up at point number three, so plant a flag right here. We'll come back to it.
But for those who care to follow along, this is some really fun stuff that can get into why we want to match our cells properly in terms of their outputs. So the total current output-- this is the ideal diode equation without all the fancy two-diode model, recombination of the space charge region, recombination of the bulk, without the series resistance and shunt resistance. Just a very simple explanation of this ideal diode model.
What we're doing is our M is the number of cells in parallel and our N is the number of cells in series. So we go back to our equivalent circuit diagrams where we have two voltage current sources that are connected in series. If you connect those two in series, now the voltages will add. So the effective voltage across the entire system, if you draw a black box around your two individual voltage producers, the effective voltage across the entire system will be voltage 1 plus voltage 2, in series.
The currents-- now, if you think about the current, you can think of current-- the first order-- as a river of electrons trying to flow through your circuit. If there's any bottleneck somewhere, that's going to limit the current that can flow through the entire circuit. So the combined current is going to be limited by the worst performer. In mathematical terms, it would be the harmonic mean. 1 over effective current would be 1 over current 1 plus 1 over current 2.
So what we see here is, if we have a number of cells connected in parallel-- now, instead of having them connected in series like this, you connect them in parallel-- now the currents are adding. And the voltage will be limited by the worst performer. Because the potential across both is going to be limited by whatever the lowest potential is.
So when we connect in parallel, we add currents. And when we connect in series, we add voltages. That's a very simple way to think about it, and that's why we have the M appearing here up top. If you have a number of cells connected in parallel, you will essentially be multiplying the [? illuminated ?] current of one cell by whatever M it is. And if you have a number of cells connected in series, that will impact the voltage.
OK. So that's an expression that is useful for N and M number of identical cells connected in either series or parallel, respectively. And in practice, if we have bad performers in the bunch, if we have one cell that's performing worse than another, either because it is intrinsically worse-- it is a defective cell that somehow got in there or became defective during use-- or it's a temporary effect. Maybe a seagull just landed on top of that cell and is shading it. Right?
So whatever the reason is-- temporary or permanent-- that cell number 2, now, is a bad apple. And so if we're looking at parallel mismatches, we take what we know about cells in parallel. OK. The voltage is limited by the worst performer. And we look at the individual IV curves of the good and the bad cell. This is the good cell right here, this curve there. Right?
And so this, now, is positive power. Essentially, power coming out of the solar cell is plotted in the first quadrant. We have a lot of power coming out and a large voltage for our good cell. Here's our bad cell right there.
So slightly lower current outputs given by the logarithmic effect. And the voltage is lower, significantly lower, than, say, the good cell. And so the combined output right here, the current at short circuit current conditions, is going to be the sum of the good and the bad. So that's there.
The voltage is going to be the harmonic mean, so limited by the worst performer. That's how low-voltage cells can really mess up parallel strings. So for example, this set of two rows of cells, this one right here, which is all connected in series. It's connected in parallel to this one and this one. And so if one of these three subsets of cells has a lower voltage than the others, the combined voltage output of the entire module will be lower.
Similarly, now we've put them in series, if we have a bad cell that's producing less current than the good cell-- and notice that they're saying open circuit voltage now just for the thought experiment here in the classroom. Typically, if a cell is performing worse than current, it will also have a lower voltage. But just for the thought experiment right here, we have bad cell, good cell. Bad cell has about half the current of the good cell.
So the voltage output in series is going to be the addition of the two. Boom, boom. Then you're up to here. But the current output will be limited by the worst performer. And again, the combined output of this set of cells connected in series is going to be right around here. And so based on your cell performance-- if you know that your voltage has a certain variance in your manufacturing line and your current has a certain variance in your manufacturing line, that might entice you to string together your cells in one way or another, depending on how you want to mitigate your losses.
Shaded cells-- so up to now, everybody kind of gets based on Kirchhoff's laws and so forth from equivalent circuit diagrams, so we're rolling. Now we're going to get the shaded cells. And here's where it becomes a little bit more difficult to understand absent the reading in that book over there.
Did everybody get a chance to see the blue book? Did it make its rounds over here? No, it didn't. Why don't we pass the blue book around? Omar over there didn't get a chance to see it yet.
We're going to consider that we have 10 identical solar cells connected in series, and this one over here is shaded. What happens now? So this is described, I believe, in figure 5.7 in the book, one with the green little tab.
We've assumed that the combined IV characteristic of the n good cells is this one right here and the one bad cell is this right here. So it's a little bit of a strange IV characteristic. And what happens next?
If you combine the two in series and you use the same protocol that we've done so far, the voltage will add. So whatever voltage gain right here has been added over here. But the current is going to be limited by the worst performer, which happens to be this solar cell right here. And so the combined output is going to be lower-- significantly lower-- than those of the good cells. And that's what happens when one cell in a string is shaded if there aren't any fancy electronics to allow the current to bypass it.
Furthermore, this amount of power will be dissipated in the bad cell when the string is short circuited. It can change a bit when you start moving toward operating conditions, but it's a sizable amount of power being dumped into that bad cell. And in practice, that cell is reverse biased. Let me show you using our wonderful PV CD-ROM exactly why that is.
So here we have two solar cells connected in series. And they're both producing an equal amount of current because they have an equal number of these yellow arrows incident on them. And they're equal in every other aspect. So it's still a thought experiment right now.
The current that is cycling through this circuit, in the external circuit right here-- the magnitude of that current is being indicated by the color. And, in this particular case, it's very bright. It's bright green. It indicates a lot of current is flowing through it.
So, up to now, everything makes sense. This is in short circuit conditions, so there's no voltage. There's no potential across either cell. It's in short circuit conditions, and current is flowing through this circuit right here.
Now I'm going to mismatch the two cells. I'm going to exchange one of the cells for a bad apple. OK. First off, I know, just from what we've been talking about so far that the current is limited by the worst performer, that the current flowing through the external circuit is now going to be lower. And the first order-- it's more or less approximate to what the current is in this bad cell.
So the bad cell right here, which is indicated by the two arrows now instead of the four, this shaded cell right here is producing less current. And so I know, based on what we've talked about so far, that the combined current output of the entire circuit is going to be approximately this one. So hence you see a darker color represented, the same color that's flowing through the cell.
So what happens to the large amount of current that's being generated inside of this one? That cell still has the capacity to produce a large amount of current. Where is it going?
Well, some of that current is going through this cell right here, forward biasing it, which would result in a negative bias, or reverse bias, of the bad cell. Because the potential across this combined system still has to be zero. You're in short circuit conditions, so the potential here has to be equal to the potential there.
So what I've effectively done is I've reverse biased my bad cell. And we'll get to why that's a problem later on. Those who are electrical engineers can already begin conjuring up ideas of reverse biased breakdown. We'll explain why reverse bias is bad in a few.
Now, if you had a series of these cells connected-- a bunch of these cells connected in series-- and they were all producing copious amounts of juice, and you had one bad apple in your string, you would be dumping a considerable amount of power across that. Or you could be dumping a considerable amount of power across that one cell. And that's where this graph right here comes from, the power dissipated within the bad cell.
And that's a problem because if there's any weakness in the p-n junction of that bad cell and you're reverse biasing it, you're going to be flooding a lot of current through that one little spot, which means that that spot's going to heat up. And it's going to form a hot spot, which has the potential to get very hot. And we know that the encapsulant materials flow at around 150 to 175 degrees C, and some other material failures can occur above that. So hot spots are bad.
If you reverse bias your devices, you can enter what's called a breakdown regime that, essentially, is driven, oftentimes, in a real solar cell by isolated points and your weaknesses in the p-n junction where current is going to flow. And that leads to the hot spot failure I just mentioned. Here's thermographic imaging-- not using the fancy camera that we have downstairs, using a much simpler camera out in the field, lower cost.
These are high enough temperature variances to be able to detect using pretty low-tech technology. It's not a small amount of current going through a solar cell. These are large, massive amounts of current going through defective cells in the field. And so you can use these cameras to visualize the underperforming cells inside of modules. Now, that's pretty nifty.
All right. So we planted that flag, and let's come back to it. We're going to describe how microinverters and microelectronics can improve module performance output. Even if you didn't follow all the detailed explanation until now, you can appreciate the fact that a bad apple or bad solar cell, connected in series or in parallel with the rest of them, can cause the combined output of the module to be lower. And so we're going to talk about how microelectronics and eventually microinverters can help resolve that, either on a cell level or on a larger module-to-module level.
The simple principle of a bypass diode of a cell is this. If the reverse bias current becomes too large, instead of forcing the current to flow through the bad cell, you can force it to flow around a bypass diode. And therefore, it short circuits-- it leaves the cell out of the circuit. Right? The current is flowing around it, and so you result in less of a degradation of your module.
To put it from an equivalent IV curve perspective, instead of adding this IV curve here with the rest of them, you're simply moving the current around it so you would have the n good cells, and that's it. End of story. The 10th cell would just be taken out of your circuit. It would be pushed aside.
OK. So on a cell level, that make sense. Let's skip over a couple of these, I think. On a module level, as well, it can make a lot of sense. And here, it really does because the shading losses on a module level tend to be more severe. You can have, for example, a tree in the wrong place or a telephone pole somewhere, shading one particular module in an array, an array of modules. On my roof, for example, there are 12 Sharp 187s.
So if you have a series of these modules up on the roof, you can cause the current to circumvent one of the modules that's underperforming, again, using the bypass diodes. And those are fairly standard in most of the junction boxes. So inside of here, there is some very simple electronics to prevent the modules from becoming power sinks and perhaps even drawing power from the grid.
OK. So microinverters-- some people say, well, instead of just managing power on a DC basis and either dealing with the mismatch loss or cutting bad apples out of the circuit altogether, let's do something even better. Let's try to-- to put this in childhood education terms-- let's let every module perform up to their greatest potential, and then everybody is happy. Right?
So we're going to convert the power directly on the back of the module into AC, alternating current. And then we're going to use electronics from there to essentially add the currents together once we have defined the voltage at 120 volts at alternating current. And the advantage of that is that if one module is underperforming, instead of contributing zero, it's now contributing some fraction.
If the mismatch was small enough not to trip any of the bypass diodes but still degrade the power of the overall system, now you're able to recover-- you're able to allow that good module to perform up to its true potential instead of being dragged down by the others. And so the microinverter companies that are proposing, and actually marketing and selling, modules that apply the electronics directly in the back of the solar panels have the advantage of being able to eke out several additional percent of total power from the array, from the system. The disadvantage of distributing these microinverter components over every single module is?
AUDIENCE: You have to make a lot of them, and they cost money.
PROFESSOR: You have to make a lot of them. They cost money. They're costly, and-- mean time between failures? Increases. Right? Potentially. Potentially. So if you have an inverter-- how many people know how long an inverter lasts before it needs to be replaced, typically? 5 to 10 years. Now it's up to, say, 7 to 12, but yes. Somewhere in that range. Less than the 20- to 30-year life span of your array.
So now if you have these distributed little inverters across every solar panel and they fizz out after a certain amount of time, somebody has to go up there and replace them. First, identify where they went down and then replace them. So microinverter longevity is an important part of reducing risk and marketing inverters. So the microinverter community is helping to push, or improve, the quality of inverters over the entire solar spectrum, which is kind of cool. Yeah.
AUDIENCE: What's the [INAUDIBLE]?
PROFESSOR: So, my understanding from Rajeev Ram, who is actually down in ARPA-E right now-- he's a professor at MIT who's been assisting the DOE over the past couple of years-- most failure in inverters relates to the power electronics itself and the high speed switchers that are used. That's my understanding. I could look into that a little bit more and get you more details about precise inverter failure modes, but that is my rudimentary understanding from discussions with him.
Sorry, my screen is still cracked. It's going to be fixed tonight. Advantages of integrated power electronics is maximum power point tracking. This advantage is-- OK. Yep. OK. And so it's still a relatively hot topic. There was an ARPA-E call for improved power electronics across solar and other industries. And there is some discussion in the PV community as to how much to adopt this new technology, especially in residential systems.
So trends in installations in general. In the past, installations were heavily labor-intensive. There is now a large amount of pre-assembly done before the modules hit the roof, but there's still a sizable number of components that go into every single solar module. And that takes time, to mount everything out there in the field.
So what are some other-- what is the dream of any solar installer? Is to get this roll of material just unrolls, unfurls, onto the roof and using a staple gun-- bang, bang, bang, bang, bang-- or a nail gun. Everything is locked down. It's lightweight. It doesn't underperform when it gets too warm. These are all the specs that would constitute, say, an ideal solar module. We don't get that lucky. We have to make compromises in reality.
Say, for example, on my roof there was a soft spot on the roof that had to be worked around. The structural engineer had to be called back and evaluate and figure out whether or not it needed reinforcements and so forth. So we're still far away in terms of where we need to be for solar installations to really be truly low cost. And a lot of it has to do with the balance of system design.
So I mentioned a few companies are attempting to integrate the connections into this facile click-lock mechanism. There have been patents filed, I think, since the 1990s on this. Probably even earlier. More recently, some companies have marketed a product in this regard. And of course, that increases the facility of installation. There's even a few that are selling, I think, it was Akeena at Lowe's and, for a time, BP Solar was selling at Home Depot. They're examples of companies beginning to market their product at hardware stores. Question.
AUDIENCE: Could you define exactly what you mean by balance of systems?
PROFESSOR: Yes. So balance of systems is defined as everything beyond the module. So that would include the wiring, the framing, the racking, the inverter. Yes. That is balance of systems. Excellent question. Excellent question.
So we're going to talk about some of the tests that the PV module must pass to ensure reliable multi-decade service life in the field and some of the shortcomings of the tests. I'm going to motivate this by saying there's reason to be concerned, in the early days of the industry, about performance and reliability.
This was a study done by Arne Jacobson. The group that's working with the World Bank will be as well working with Arne Jacobson on the class project. He's a professor at Humboldt University in California.
During his PhD with Dan Kammen in Berkeley, he did an audit of rated versus actual power outputs of solar modules in the field of Kenya in Africa and found a rather large discrepancy between what was promised and what was actually delivered. This is a great example of field work being done-- good old-fashioned detective work-- that leads to changes in the industry. That got a number of the under-performers to improve their product.
So I mentioned there's a question of the customer. Am I getting the real value that I've been promised? Especially in the absence of independent monitoring. If you're not actively measuring the power output and recording it, there's always that question lingering. So module testing and reliability is meant to ensure product quality and output quality.
Secondly, it's safety. I mentioned that these thermographic cameras-- this is an example in an array, of a hot spot. It's not too hot right now. It's 36 degrees or so, but everything else is down around 15. If you imagine this being on a sunny day-- a really sunny day-- this hot spot potentially could be getting up much higher. So that's obviously of concern.
And there's as well hot spots or heat that can be generated from the junction box on the back. This contains a fair amount of electronics, again. And if there is some failure of the electronics inside of here, you can have the combined power output of the entire module arcing through a given component back here causing sparks to fly. And this is where this Photon International article from 2009 comes in where they say it's extremely important-- the upshot of the entire article, I gave it to you in its entirety. But the upshot is let's not play with fire, meaning let's make sure that the safety standards of solar modules are really up to spec so that the risk of something catastrophically bad happening is negligible. Because there's nothing worse for a nascent industry than to have some, dare I say it, Fukushima equivalent occur on some very high-profile event that causes public opinion to sway against it.
So making sure that modules are safe is of utmost importance to preserving the positive image of solar. And that's what this article here is meant to convey, and you're welcome to read it in detail. It cites one particular example where that didn't happen and it did lead to it a little PR event-- well-justified, in fact.
Shown here are a series of tests that one can conduct with solar modules. I showed a list of different IEC tests, standardized protocols. These are a series of tests, typically between 12 and 20, that represent a battery of tests for the solar module. And if they pass it, they are IEC certified.
And you'll see on the back of certain spec sheets, like this one from First Solar right here, the different testing agencies that have certified the module, these little logos represented. So it says it's UL certified, CE mark, certified according to IEC spec 61646. That's the one right up there at the top and so forth. So certification is very important.
What happens during testing? Well, we'll see it because when I designed the Fraunhofer CSE labs, we designed it, Roland Schindler and I, with the IEC tests in mind. So each of the pieces of equipment out there are to test one component of the IEC test.
Included in the test is environmental cycling, where you take a module, stick it inside of what looks like an oven. And it heats it up, cools it down, heats it up, cools it down or maintains a high temperature with high humidity. Different types of environmental chambers do different things, but they're meant to simulate an accelerated aging process. Again, since most diffusion processes are driven by Boltzmann statistics, there's an exponential increase with increasing temperature. And hence, we can simulate accelerated degradation at higher temperatures.
So it's not uncommon that you hear about 85/85 tests, which mean 85 degrees Celsius at 85% humidity. So that is a massive jungle. We don't have, thankfully, places on earth like that. The maximum temperatures are at around 45, 50, 55 degrees C typically and, in those cases, very dry.
But in here, 85 degrees Celsius-- it's still below the boiling point of water. Very high humidity and the humidity, if there is any place for the humidity go inside the module, if you have any failure of your encapsulant material or it can get inside, it will. And it will begin degrading your module. You'll see the power output decrease. And after three months subjected to that sort of torture, if the module is poorly designed, oftentimes it will fail.
Now, some of the shortcomings of these tests-- that humidity cycle isn't necessarily, with the module, experiencing an applied bias voltage. So if there's any electromigration affect, you might not detect it with that. So there are people designing new forms of tests that will probe other failure modes, other degradation mechanisms, that aren't necessarily captured in these IEC tests. Do you have a question? No. No question. OK. Yeah. Over here.
AUDIENCE: What is the failure mode of degradation by water?
PROFESSOR: By water? Yes. So water, humidity-- typically what happens is, in the laminate itself, there will be some part at the edge that water can get in. Not always, but in the poorly designed ones, yes, and so the water can get in and begin diffusing into the module. So if you have a thin film material that reacts with water, you might degrade the actual absorber layer itself.
If you have a silicon device, the water could react with the metal and cause it to corrode-- cause it to oxidize, in other words. And of course, in reacting with the ethyl vinyl acetate, it can cause the encapsulant to delaminate from the cells and tear apart. And so you see this discoloration of the module.
Those are some of the failure modes that can occur when water gets in through the side. If it managed to permeate the encapsulant materials themselves, those sorts of effects can occur directly as opposed to coming in through the sides. Another question.
AUDIENCE: So they presumably test some small fraction of the modules.
PROFESSOR: Yes.
AUDIENCE: That they [INAUDIBLE].
PROFESSOR: Yep. So they presumably test some small fraction of the modules. And then, if those modules pass the test, which take several months to conduct-- and that's why there's a large barrier for module innovation-- but if they pass those tests, then they assume that every module-- yes. So there are a series of, I would say, definitions of what constitutes a major alteration to the process. So if you majorly alter your process, then you have to get your module recertified. But if you stay within those bounds, then you can continue selling under the old certification.
It's not too dissimilar to the way our food is tested by the FDA. It's not every single hamburger. It's a spot test. In this particular case, these are examples of the newest technologies to come off the line, and you get a couple of chances to pass. If you fail the first one, it's not the end of the world. It just means that you have to pass the next time or else you have a stain on your record.
And many of the companies now have their own facilities to do these tests in-house. They still send them out to the certified testing bodies that are independent to get the final seal of approval. But they know fairly well before they send them out, are we going to pass or not? In the early days it was-- you always had to hold on to the seat of your pants because you never quite knew what was going to happen when the test results came back. But now it's much better, much more predictable.
And my favorite test out of all the ones in there-- there are a number, if you imagine a dozen to 20 tests, from illuminating it and measuring the power output. One of my favorite tests involves a hail gun. So in a similar manner to how the FAA tests windshields of planes and plane engines by shooting frozen chickens or turkeys at them, in this particular case, we're shooting frozen balls of water at the modules and seeing if they crack. That's one of the ones.
You can envision, as well, an immersion test underwater combined with bending to see if there's any failure in the module. So they are tested quite extensively using these protocols. And the objective is to ensure quality and safety to the consumer.
AUDIENCE: What's the top right test?
PROFESSOR: This top right? Oh, top right over here? This is a mechanical loading test, what it appears to be, where you just exert what is typically-- that's what it appears to be in this particular case. I could be wrong. But my understanding is that this module is constrained on the edges right now and that there's a load being pushed down in the center.
You could also envision a sandbag test where you load physical sandbags. There are a variety of different types and variants. Yep. OK. So that comes from the First Solar spec sheet.
Describe the differences between various types of PV systems. Since we're running short on time, this is a bit of a repeat from what we talked about the very first day in class-- the non-tracking, the tracking. So I'm going to fly through that into grid-tied and stand-alone.
So grid-tied and stand-alone systems is very straightforward. If you have a system that is tied to the grid, somehow it has to be able to interface with the grid. Let me go to that in a second. Here.
So if you're having a grid-tied system, that means that your house or solar array, whatever it is that's producing the electricity, has to be tied to the grid and interface with it. Since the electricity is alternating current, there has to be a device to convert the direct current power into alternating current. And that's called the inverter. We've already touched upon that a few times over today's class.
And these objects range in size. For the ones in the house, you could find inverters that are about yea big that can handle a few kilowatts. The ones that are handling these large solar field installations, or segments thereof-- let me find a large solar field installation.
Here we go. Here are some pictures of large solar field installations. This is a road. These little green dots are trees. That's a big field installation right there. Those inverters are warehouse-- can be much larger sized than the smaller ones that you'd find in your house.
And so, listing the major balance of system components, the PV array would be connected to the grid by an inverter. The excess power would be sent to the grid, causing the meters to spin backward, which is a very gratifying observation from a homeowner's point of view or, at the very least, a rate payer, utility payer's point of view.
The act of sending the power back to the grid is a bit of free riding because I'm not paying for the grid. I'm a consumer, but I'm getting credit for the electricity that I put back in the grid. But I'm not helping to maintain the grid, necessarily. I'm paying some transmission and distribution costs on my utility bill, but that's essentially the same cost that everyone else is paying.
Utility companies have begun getting wise about that and have begun leveraging surcharges based on the input of solar electricity into the grid, which I now pay. So I'm no longer a free rider. I was for a little bit. So, mounted onto the roof or the ground. If you mount the solar panels, you need racking and framing materials. That's also balance of systems.
OK. So. Check. Check. Check. Check. You typically have, as well, a circuit breaker, or just a very simple lever that disconnects the solar panel from the grid. Why is that important?
The utility companies know that, on my house in Cambridge, there's a solar array and that that array is connected to the grid. Let's imagine a tree falls on the power lines during the next storm, and the utility crew has to go out there to repair it during the day. They know that, although the rest of the grid is down, there's that one little power center on my house that's injecting power into the grid.
And so they'll come along to my house, disconnect the solar panels from the grid. They'll go to my neighbors, disconnect theirs as well. Go to the person across the street who also has solar panels, disconnect them. Then go to work on the utility line. So that's why the external circuit breaker is very important.
Back to inverters. Modern efficiencies of the inverter component range between 94% and 97% typical. Record inverter efficiencies up to 99% have been demonstrated. And they all have what's called maximum power point tracking, which means that they will be able to sense what the combined output is of the array on your roof and adjust the resistance in the circuit to match the maximum power point.
And that way you're producing the maximum amount of power that you could be, potentially, under any given illumination condition. So a lot of fancy electronics goes into the inverter. I really have to give them a lot of respect in that regard. Typically five to seven, maybe even 10-year manufacturer warranty.
And the mounting methods-- we talked about mounting and racking. This type was fairly common in the early days of solar where you'd have these field installations, especially built on hills in California. I would say most common now is really just mounting directly on a roof-- oftentimes, flat. For large field installations, one-axis trackers are very common nowadays. Tracking as a function of time of day and then manually adjusting the entire array for season, if need be.
So the grid-tied systems causing meters to spin backward. Again, very gratifying. And this is an example of my utility bill, where you have a balance of $163 negative, so they're giving me credit over the course of a year. In the winter time-- this was an example of a month in December when a lot of snow happened and the panels were covered for quite a while, or at least partially shaded by the snow for a while. And we drew power from the grid on net that month.
But on all the other months we, on net, produced power. And negative numbers aren't shown in the scale here. But if they were shown, you'd see probably greater negative value in the summertime when others were producing a lot of power in the summer-- more sun.
And at the end of the year, they draw a line at the bottom. And they say, did you owe us money? No? All right, great. If we owed you money-- tough luck. If we're zero, then everybody's happy. So we typically produce more electricity than we consume in our house. And that's an example of what happens on a practical level.
Off-grid grid PV systems, just ever so briefly. So let's imagine that you're running some illicit business, and you want to be off-grid. Or let's imagine that-- instead, a more realistic example, let's say that you're afraid of the next power outage, of being out of power for the next 7 to 10 days after the storm passes through your house. And you want to isolate yourself from the grid, if need be.
You would want to have a charge controller that would be able to distribute the power to the DC loads, or to batteries to store them, and then the inverter, going through the AC loads or to the grid thereafter. So it is possible to architect a system that is semi-autonomous. But it does require more components and more cost.
And I just wanted to emphasize one thing as we close up. Life is really good as an installer right now. If we flashed back to four or five years ago, life would have been really good as a solar panel producer, especially if you were involved in the feedstock industry.
But now the prices of modules have been severely squeezed by low-cost competition from overseas. And the pricing of modules is around $1.03 per watt peak. This is some of lowest price modules that are out there right now. And the price of installing the system on your roof here in the US is around $5.20. Who's pocketing the $4.20? Who gets to keep that money? Is it the installer or the module producer?
AUDIENCE: The installer.
PROFESSOR: It's got to be the installer. Right? And who's suffering right now in the industry? No matter where you are-- US, China, Germany. Who's suffering right now? The module producers. The people manufacturing this stuff right here.
All right. Which brings me to my current topic of the day. Everybody picked up this one right here, this Greentech Media article? This is really current. November 8, that was two days ago. Eric Wesoff. He's higher up there in Greentech Media.
And he's writing about the China-US trade war that's evolving pitting-- within the US-- pitting the module manufacturers against the installers. So the installers, these guys who are pocketing a lot of money right now, are saying no, no, no, no. Let the Chinese modules come in. It's great. It keeps our costs low.
The folks who are manufacturing the modules, on the other hand, are saying, well, wait a second. They got unfair treatment by their government. And the Chinese module manufacturers, in turn, are saying, well, it's not only us who are getting subsidies from the government. Look at yourselves. You got subsidies, too. And US manufacturers say, well, you got more subsidies than we did.
So it's evolving into a little bit of a complex situation. There are some people trying to cut through all of that and say, with very simplistic messages, free trade. Or certain other people saying, made in USA is important. Or other people saying, this isn't fair for one reason or another.
But it's important to really understand the complexity of what's going on in the context of a dynamically changing market. The market is changing quite a lot. In the past, the module prices-- and I say past, recent past, as in a year ago-- module prices were upwards of $2.50, $3.00 per watt peak. It's only in the oversupply condition created within the last year and a half where the module prices have dropped precipitously down to $1.00. And that happens when you're in an oversupply condition, whereas these installation prices really haven't budged too much. They're enjoying the fact that it's in an oversupply condition and there's still a market for the modules.
So it's complex. We'll explore some of the complex issues in our next few classes, and we'll dive into that in detail. I'll leave, for you to study on your own, life cycle analysis. It's very important.
Life cycle analysis-- in a nutshell, it really depends on what you consider in your analysis, where you draw the box. And at the end of the day, module recycling is going to be important as well for determining the ultimate impact, both in terms of CO2 impact, energy payback, and environmental impact of PV in the long run. So I'll leave you with those thoughts. And we'll see us on Tuesday.
Modules, Systems, and Reliability (PDF - 2.7MB)
Supporting Materials
Articles referenced in this lecture:
- Wesoff, Eric. "Solar Module Pricing: $1.03 per Watt for c-Si." Greentech Media, Oct 14, 2011.
- Campbell, Carolyn. "US Has an Average Solar System Price for $5.20/W." Greentech Media, Oct 17, 2011.
Free Downloads
Video
- iTunes U (MP4 - 229MB)
- Internet Archive (MP4 - 229MB)
Subtitle
- English - US (SRT)