Flash and JavaScript are required for this feature.
Download the video from iTunes U or the Internet Archive.
Topics covered: Stem Cells/Cloning 1
Instructors: Prof. Robert A. Weinberg
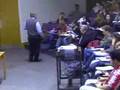
Lecture 29: Stem Cells/Clon...
Good morning, class. Nice to see you here.
Yesterday morning I was in Australia and today I'm here.
And it's nice to be back. It's a long way home.
We're talking today about the issues of growth and differentiation about normal cells becoming specialized into different types of cells throughout the body and how that leads ultimately to the issues of organismic cloning. And, to give you an overall background about this, I want to show you the way that a worm is put together. This is the worm C. elegans.
I don't know how well this overhead will come out.
You can see it reasonably well. So, this is the worm Caenorhabditis elegans. It's been an object of much study here.
Bob Horowitz in our own department just got the Nobel Prize for his work on this.
And the reason it's been the object of so much study is, in fact, that it's a relatively simple organism.
It's the way that you and I looked about 600 million years ago when Metazoa, remember Metazoa means multicellular organisms, first arose on the face of the planet. Let's see if I have too much overlap here. Well, that's about the way it should look. Now, what's remarkable about this organism is it has something like 965 cells in the adult. The cells all descend from a fertilized egg. It happens to be that this worm is a hermaphrodite. So, you probably know hermaphrodites are both male and female and it can fertilize itself or two worms can get together and fertilize each other.
Because, as you can see, it makes both eggs and it makes sperm. And what's most interesting is the finite number of cells in the body of the adult. As I said, it's 965, whose lineage can be traced through a pedigree that's given right up here. So, one can plot out, with great precision, how all the cells, how the egg cell and the fertilized egg divides in two, each of those cells divides again in two and how ultimately one has a whole series of different descendents from different branches of this very elaborate family tree. Now, one of the issues that we want to pursue is the fact that the genomes, in principle, all the cells in this organism are the same. That is to say whenever the cell goes through a cycle of growth and division the cell is genetically identical, yet phenotypically, yet the behavior reach of these cells becomes increasingly different.
And here you can see the different lineage of cells.
This organism devotes a disproportionately large amount of its anatomy to reproduction, much more even than we do. Here, this is the cuticle, the outer coat. Here's the vulva, the female organ.
This is the male organ and a variety of other cells types.
Here's the pharynx. And yet all the cells in all these organisms, with the exception of the gametes, remember gametes means sperm and egg are genetically identical. The gametes have only half the genetic content. Everybody else is identical, has a diploid genome, that is to say two copies of each gene.
And one can trace this all out. And this represents one of the great mysteries of developmental biology, which is to say how are cells that genetically identical to one another genotypically identical to one another, phenotypically quite different one from the other? What makes them so different?
In fact, this image that we have here, which in itself represents a stunning achievement, that is being able to trace the pedigree of each cell in the adult body is very different from our own lineage because, as I may have mentioned to you in the past, each of us goes through ten to the sixth mitoses in a lifetime, you and I. That's ten to the sixteen cell divisions. And at any one time we have roughly three times ten to the thirteenth cells in our body. So, that means, if you think about that carefully, the ratio between these two suggests there's roughly a hundred times more here than here, three hundred times more It means, roughly speaking, that our body turns over roughly a hundred times in our lifetime.
That is to say the cells turnover. Not all of the cells, but that there's a continuing replacement of existing cells with new cells. After all, if, in fact, there were no such replacement than we might, as an adult, be formed of this many cells, and we would stay with the exact same number of cells throughout our lives. But this, in fact, is the number of cell divisions. And so, there's an enormous turnover which, right away on its surface, independent of the fact that it's an enormously large number, precludes one from really being able to draw out a pedigree like this, prevents anyone from really understanding how each particular cell can trace its line of decent back to the fertilized egg. So, what we really want to explore, in this lecture and the next one, is this major puzzle that one starts out with a fertilized egg. Let's say this is a fertilized egg.
It divides in two. It divides in four. And through subsequent cycles of growth and division we ultimately end up with the adult.
Already at the four-cell stage, here in a vertebrate embryo, these cells have begun to take different phenotypic paths.
That is to say cells have begun to commit themselves into entering into one or another differentiation lineage. And when I say a differentiating lineage, I mean a group of cells which has already made the decision to become blood cells, to become gut, to become nerve cells and so forth. And these commitments already start here at the four-cell stage, and they continue to play themselves out until one reaches a newborn, and then thereafter one just grows bigger. One other important thing to show here is that this pedigree that I showed you here is not simply the result of exponential expansion of all the cells, because many cells, during the course of development, are actually weeded out from embryonic tissue. And this happens even in our own development. For example, here, if we look at our fingers, I remember now I talked about fingers about two weeks ago and got myself into some hot water. If we look at our fingers, you'll see here we have five fingers, God willing, and but early in embryogenesis our hand looks like a solid flap of tissue.
And what happens, during the course of vertebra development, is that the tissue in between the beginning fingers is eliminated through the process of apoptosis. Apoptosis means programmed cell death. Apoptosis is equivalent to cell suicide. And what I mean by that is to say that development involves not only the exponential proliferation of cells but it involves the selective elimination of cells here in a very obvious anatomical way.
It sometimes can be defective, in which case individuals are born with large webs between their fingers. And this is part of normal development. And the same can be said here. If there were no apoptosis during the development of this worm embryo then there would be vastly larger numbers of cells. We mentioned implicitly apoptosis during the development of the immune system, because recall there that bee cells, which are destine to produce antibodies, if they produce inappropriate kinds of antibodies, if they produce antibodies that are self-reactive, i.e., recognize some of the body's own proteins, those cells are eliminated by apoptosis. If they produce defective antibody molecules, they're eliminated by apoptosis. And, therefore, differentiation, which is what we're talking about here, involves not only the commitment of cells to a certain lineage, but the purpose of elimination of cells in certain parts of the organism in order to carve and sculpt out properly shaped tissue. Again, our fingers are one dramatic example of that. It turns out we can learn an awful lot about this process by studying one specialized adult tissue, which is to say the organs of hematopoiesis.
And they'll teach us a lot about some of the lessons we need to learn about organismic development and differentiation.
And when I use the word hematopoiesis, the term hematopoiesis, or the adjective hematopoietic refers to the creation, the formation of different kinds of blood cells. In fact, we know that all the cells in the blood descend in the organism from a common progenitor.
And this progenitor is called a pluripotent stem cell.
Pluripotent means that this stem cell, and we'll define a stem cell momentarily, this stem cell is able to create descendents which can commit themselves in a number of distinct directions.
They can differentiate in a number of distinct directions.
In this case, we see all these various kinds of white and red blood cells which descend from this pluripotent stem cell.
And, as a consequence, we call it pluripotent because it has these multiple distinct types of differentiation lineages.
So, here we talk about pluripotent. Later on I'll talk about totipotent cells. Totipotent are cells that can do everything.
Therefore, in fact, what's a totipotent cell?
Well, a cell in the early embryo, including a fertilized egg is totipotent in that it can direct its descendents into all of the differentiation lineages in the body. Here we have a cell that's already more limited. It's only pluripotent, pluri in the sense of multiple but not total. Totipotent obviously means it can do everything. And here we see the different kinds of derivative white blood cells that exists in the bone marrow and in the circulation, and there's a whole series of different ones of them.
We'll talk about some of them shortly, but we've already encountered some of them up here in the form of T cells and B cells.
When we use the word stem cell the essence of the definition is as follows. A stem cell is a cell that can self-renew and it can also have a differentiated daughter.
So, here's the way one can diagram a stem cell. Here's a stem cell that has two daughter cells. One daughter cell is exactly like Mom and the other daughter cell has undertaken a program of becoming differentiated. So, here we have an asymmetric cell division on the part of this stem cell up here.
We'll prove later on that these two cells are genetically identical, but clearly they're reading out their genes in quite different ways. This cell is absolutely the same as the mother cell. This cell has already committed itself. It's made the commitment to differentiate in one or another lineage. And another way of noting this graphically is the following. We can draw a picture like this, as we did before, and here we'll have a second arrow that goes around like this. It loops around back on itself, and that implies the whole program of self-renewal. Now, the whole concept of self-renewal is a simple one. If a stem cell can self-renew, that implies that the process of growth and division does not deplete the pool of stem cells in the body or in a particular tissue.
So, let's imagine what we're looking at here. Here we have a stem cell.
It has one cell that is just like Mom. This is a differentiated cell.
Once again, you can have a growth and division. This is, once again, a cell like Mom. This is a differentiated cell and so forth. And what you notice here in this arrangement is that the stem cells perpetuate themselves. They are self-renewing.
And, as a consequence, the pool of stem cells is never depleted in the best of all possible worlds. It turns out that in most of the tissues of our body there is self-renewing stem cells going on, because most of the differentiated cells in our body have a finite lifetime. Not all of them but most of them. And when I say finite, I mean it can be measured in a matter of days or weeks or months. In the case of the brain, things turnover very slowly. Even in the case of our bones, our bones actually turn over roughly once, 10% a year.
10% of the matter in the bone is actually turnover in every year.
So, almost all tissues in the body are in a process of continuing self-renewal and repair. And that self-renewal and repair is maintained by this stem cell compartment, as is indicated here.
This has certain kinds of great advantages, and one of the advantages is indicated by the following. Let's imagine that we draw a picture, just for the sake of argument, of one of the most highly proliferative tissues in the body, which is to say the lining of the colon or of the duodenum.
So, here we have, let me draw it slightly differently, here's the way the lining of the small intestine looks like.
Out here are the contents of the small intestine.
So, let's say here is the lumen of the small intestine. And here we have, protruding into the lumen, when I talk about a lumen I'm talking about the bore or the channel of a cylindrical or tube like organism.
So, here is the lumen of the small intestine. Here are these fingerlike projections, they're called villi, that protrude into the lumen of the small intestine. And down here at the bottom of this are these cavities that are called crypts, C-R-Y-P-T-S. These are the crypts. Now, what's important to realize is that what goes through our intestines is not that pleasant.
It's pretty corrosive stuff. I probably told you this already, more bacteria living in there than we have in our entire cells in our entire body. There are all kinds of digestive juices.
And so the cells out here at the tips of these villi are continually exposed to all kinds of corrosive material, including the junk that we eat everyday which is flowing by like this. And this indicates how critically important it is that we have self-renewal, because the cells out here, being continually exposed to the most corrosive kinds of influences, are rapidly damages.
And, therefore, the cells out here have a lifetime of only three or four days and are then induced to jump off the end of a gangplank and commit apoptosis. So, the cells at the tip of the villis are continually jumping off and dying. And what's happening is that down here in the bottom of the crypts we have stem cells.
The stem cells are continually producing progeny that have committed themselves to differentiate. And the progeny, as you might guess from what I've just said, are continually migrating up the sides of the villis up to the end here. And this whole migration takes four or five days, and by the time they get to the tip and have stuck their heads into the contents of the lumen of the intestine for that period of three or four days.
Finally, they're eliminated and they jump off into the abyss.
So, there's a continuing action going on here.
The stem cells are continually dividing. And what advantages does this have for us? Well, it means that cells that are damaged are not allowed to hang around for a very long period of time, i.e., cells up here in the top that are exposed to, for example, potential mutagenic influences are rapidly eliminated.
Why is that good? Because the mutagenic influences up here could well create a mutant cell that, in principle, is able to become cancerous. And the body says, well, I don't mind if that happens because these cells up here are going to be eliminated anyhow. They're going to be pushed off the end of the diving board or the gangplank into the abyss, so they are continually undergoing apoptosis, not as a pathological process. As a normal process. They're continually being pushed out here. And what that means is that the cells down here, in the bottom of the crypt, are actually physically protected from the contents of the lumen of the small intestine because some of the cells in this crypt are continually secreting a kind of mucus in this area right here.
It's called a mucin. And this mucin here creates a physical barrier, so the cells that are in the bottom of the crypt are never directly exposed to the contents of what's flowing by in the small intestine. And that is extremely important because, in fact, it means that these cells down here are shielded from the mutagenic influences of what might be present in the lumen of the small intestine. In theory, one might be able to evolve cells that don't mind being up here in the lumen of the small intestine. But, in fact, that's never been possible.
That is to say evolution has just said, well, we can't really evolve cells that are resistant to the corrosive influences of what happens in the small intestine. And, therefore, we're just going to use these cells for a very short period of time and then get rid of them. What that also means is the following. The stem cells down here stay within that crypt.
They don't migrate out. They stay there in that shielded site. And, in fact, if you think all this through, it's very important to protect these cells from becoming mutated because if they do become mutated they could become the precursors of cancer cells. If these cells become mutated out here, it doesn't matter because they're going to die anyhow.
And so, we now have the following kind of dynamic.
Here's the stem cell. I'll draw it again. I'll just abbreviate it stem cell. And one of the ways by which we want to preserve the genetic integrity of the stem cell is to insure that the stem cell divides as infrequently as possible. Why?
Because the whole process of cell division is itself a fallible process. Every time a cell grows and divides, as we learned from the cell cycle, there's the possibility of different kinds of genetic disasters happening in which case we might end up with a mutant stem cell.
And that mutant stem cell could in turn, I argue, become involved in creating a tumor. So, we have the following kind of arrangement. Here is the stem cell. It has one daughter that has committed herself to differentiate and the other that remains a stem cell. Well, you're saying this enormous amount of activity must involve a frenetic amount of cell division on the part of the stem cell. But that's not really the way it happens because this daughter cell undergoes a series of exponential divisions, I can't fit them all on the blackboard here, and might even yield a hundred descendents which then become the ultimate differentiated cell. This daughter cell becomes one stem cell. This daughter cell undergoes these exponential expansions in a process of creating a population of cells that are called transit amplifying cells.
And at the bottom of this hierarchy, I can't draw all hundred, there may be a hundred of these cells, ten to the second, and these cells then differentiate.
At the bottom, the hundred cells at the bottom, they go into the last stage of differentiation.
They become the specialized cells that line the tips of the villis.
Now, why is there this arrangement? Well, look at what the advantage of it is. The stem cell has just divided once, but this cell has generated a hundred progeny. And that means that the stem cell doesn't have to divide that often. The stem cell can divide once.
Every time the villis needs a hundred new cells it needs to divide only once. And, therefore, the stem cell actually is one of the most slowly dividing cells in the entire gut because it only needs to divide episodically. Each time it divides it generates this enormous array of progeny. One other aspect of differentiation, and when I talk about differentiation here, I mean the acquisition by these cells of all of the traits they need to line the colon. When I talk about differentiation in the skin, I talk about the ability, the acquisition of the cells of becoming fully competent, fully functional skin cells.
The same thing with neurons in the brain. And one additional important concept I'd like to introduce is that when cells differentiate they often become post-mitotic. Post-mitotic means that these cells give up the option of ever dividing again. In other words, as they acquire more and more specialized traits they say to themselves now I'm a nerve cell, now I'm a cell in the tip of villis in the intestine, now I'm a muscle cell, I'm not going to divide ever again. And this is generally true. The most differentiated cells in the body in general end up losing the ability to divide. They become post-mitotic.
They've exited irreversibly from the cell cycle.
They can't go back in. As you recall, we talked about cells going from G0 back into the active G1 phase of the cell cycle.
That's a reversible exit from the cell cycle. Post-mitotic cells are irreversibly committed never to divide again. And, again, that holds true for almost all cells of the body.
One exception to that is, interesting enough, in the liver.
Because what you can do with a mouse, or even with a human, is you can cut away a couple lobes of the liver, major league surgery.
And when you do that, what happens is that all the remaining cells of the liver, and these remaining cells in the liver are called hepatocytes, these hepatocytes, which until that time had been highly specialized differentiated cells in the liver, many of them divide, they double again. And in short order one ends up with a liver which is exactly the same size as one had before.
And that's actually quite remarkable because there are very few organs in the adult human being where that will happen.
There is, by the way, an interesting puzzle here, and that is the following. Let's say you cut away half the liver and many of the hepatocytes, which were already highly differentiated, began to divide again, so they were not serious post-mitotic cells, they could reenter into proliferative phase.
How do these cells know when to stop dividing so that they end up regenerating a liver of exactly the right size? People have been looking at that for 30 or 40 years. Nobody has any idea why. Why doesn't the liver when all these cells divide become one and a half times the size of its former diameter or half the size?
Nobody really understands that. In any case, I just want to indicate that there is this dynamic between differentiation and proliferative capacity, one in opposition to the other.
Well, how much hematopoiesis is taking place here in our bone marrow, for example, where a lot of this takes place? So, here are just some interesting numbers. There are roughly five times ten to the twelfth red blood cells -- -- per liter of blood.
And red blood cells, you may recall, are called erythrocytes.
Remember, it's never good to use a short Anglo-Saxon term if you can use a long complicated Greek one. And each of these red blood cells has roughly a lifetime of 120 days. That is to say after it's made it sits around in the blood for roughly 120 days.
It gets warn out. It gets gobbled up by the cells in the spleen. Much of the contents are recycled. The pigment in our stool comes from the recycling of the hemoglobin, the iron and the hemoglobin, and we're constantly renewing that.
Well, the average human being, let's say, has roughly ten liters of blood per person.
And if you put all those numbers together, I once calculated that the number of red blood cells that is being generated in the body is roughly ten to the tenth per hour. And you can go through the calculations if you want, it is fine with me, but take my word for it's roughly what's going on there. Ten to the tenth per hour.
Each time I utter a word there's probably, I don't know, ten to the seventh new red blood cells being made in my bone marrow.
So, this is not minor league proliferation.
Now we begin to understand why there are ten to the sixteenth cell divisions in the lifetime of a human being. In fact, a great majority of them, to be fair, are occurring in the bone marrow and in the intestine. And if you have an individual who's being exposed to certain kinds of chemotherapy, chemotherapy, as you may know, is very toxic for dividing cells, and the side effect toxicity of anticancer chemotherapy is largely felt, first of all, in the bone marrow where individuals tend to become anemic. Anemic means they have lower than normal numbers of red blood cells and also they lose a lot of the lining of the intestine which creates all kinds of also unpleasantness as well. So, we're not talking about something that happens on rare occasion in the life an individual. This is a staggering amount of mitosis that's happening every day of our lives. Let's go back for a moment to this diagram here and realize that when I'm talking about erythrocytes, I'm only talking about one of the branches of this multi-branch pathway. And here we see some other interesting aspects of what's going on here, and I'll give you some proofs very shortly that this actually is what it says it is.
It actually is organized this way. The pluripotent stem cell is capable of self-renewal, and it can spew off daughters which actually can go in two different directions. Its daughter may decide that it might become the precursor of the lymphoid cells in the blood or it might commit itself to becoming a myeloid precursor.
So, that's already the beginning of a bifurcation.
These cells are not yet differentiated.
They've just made the commitment that each of them can, in principle, become the ancestor of highly differentiated cells.
And these cells, we can imagine, are transient amplifying cells in the sense that even though they're committed to create progeny of one sort or another they themselves are not yet fully differentiated. Keep in mind in the context of the crypt these transient amplifying cells are on the way to becoming fully differentiated, but only at the bottom of this exponential expansion of cells do we have cells that are fully entered into a highly differentiated state. Here we see that these two cells are also stem cells in the sense that the can self-renew.
They have a limited self-renewal capacity, but they can self-renew.
And then they begin to create progeny which themselves can undertake several distinct alternative differentiation paths.
So, the lymphoid cells can become the progenitors of the T lymphocytes and the B lymphocytes. And, in fact, if you recall our discussion of immunology, there's actually several different kinds of T lymphocytes and B lymphocytes. So, this pathway has further radiations further down. Here, alternatively, these are the myeloid cells. And myeloid refers to the bone marrow. And the myeloid cells can become these kinds of cells up here, eosinophils and basophils and neutrophils and monocytes, and this class of cells is largely involved in gobbling up infectious agents and as agents which are able to defend us largely against bacterial infections. Here's the macrophage.
We talked about the macrophage. Remember the macrophage was this glutton, this pig which wandered around our tissues and gobbled up whatever kind of material it could find and presented it to the immune system. And here are several other of the lineages.
Here is a megakaryocytic and this is an erythrocyte.
What's a megakariocyte? Well, it has a very large nucleus.
That's what the term implies. And what happens to the megakariocyte is it buds off little chunks of cytoplasm lacking nuclei.
And these little chunks of cytoplasm become the blood platelets.
Blood platelets lack nuclei. They're enucleate because they're just little bags of material which are sent out into the circulation.
And, as we said also earlier in the semester, once the platelets are in the circulation they are there ready to help should there be any kind of wounding, any kind of hemorrhaging occurring.
And the platelets can release upon being activated in a site of wound coagulation factors and growth factors for the regeneration and reconstruction of wound sites. And, finally, here are our friends the erythrocytes. So, here we have a whole sequence of different kinds of differentiation commitments which are going on at an enormous rate. How do we know that there actually is a pluripotent stem cell? What evidence can I provide you that this actually exists or it's just a figment of my normally florid imagination? And the most direct demonstration of that is, in fact, the use of bone marrow transplantation.
So, when we talk about a bone marrow transplantation, or BMT as it's called in the trade, -- -- one can do a relatively simple experiment. You can take a mouse or even a human and you can irradiate it rather heavily. And if you irradiate it under the right conditions you'll actually be able to kill off all the cells in the bone marrow without killing off the mouse or the human being.
In fact, there are drugs you can also use in human beings to eliminate virtually all the cells in the bone marrow.
And then what you can do is you can take bone marrow from another organism, from another mouse or another human, and you inject it into the blood of the irradiated mouse or human.
And the bone marrow cells, many of them will home to the bone marrow. In other words, you're injecting the bone marrow cells in the general circulation, but within a couple hours they'll all end up in the bone marrow, in the space in the middle of the bone because there are many kinds of cells which have this homing capacity. They go to the right place in the body.
So, they can home. The injected cells can home to bone marrow. And then, if things are going well, these injected bone marrow cells will begin to proliferate and they will ultimately regenerate this entire cascade of differentiation decisions, as is indicated here. And, therefore, that individual or that mouse will actually be rescued. Because in the absence of such a rescue an individual will rapidly die.
You can't live for very long in the absence of an active bone marrow because these cells here are rapidly depleted. They turn over with some speed. The red blood cells hang around for 120 days we said and, therefore, you don't need to make them immediately because there's a whole bunch around that have a rather slow turnover.
But the platelets only have a lifetime of several days before they're lost, they're turned over. And if you don't have platelets you're in very bad shape because you start hemorrhaging all over the body because, remember, the platelets are there to stop up all the holes in the dike to prevent bleeding.
These cells here are very important, the eosinophils, basophils, neutrophils, and even macrophages in preventing bacterial infections.
And in the absence of having these on site one can readily succumb to overwhelming infections. Keep in mind that the reason why we're not constantly dying from bacterial infections is not because each of us takes an antibiotic pill every day, it's because these cells are on watch to kill any bacteria that happen to be in the wrong place in the body outside of the lumen of the gut.
And consequently the question is always can one rescue a mouse or a human rapidly enough? Can one replace its bone marrow rapidly enough so that this disaster from losing all ones bone marrow doesn't overtake one and the organism dies before the bone marrow has had a chance to become reconstituted, regenerated, reconstructed. Still how do we know from all this that, in fact, there is a pluripotent stem cell?
If you listened to everything I said correctly you could say, well, there isn't such a thing as a pluripotent stem cell.
There are these other kinds of stem cells, this one and this one, or these might all be stem cells. And when I'm injecting the bone marrow of a donor animal into the recipient, I'm injecting a whole mixture of different kinds of stem cells here each of which then goes on and populates a specialized compartment in the bone marrow or in the blood. So how do we know there's one pluripotent stem cell? One way to prove this is the following. Let's say we take the bone marrow from the donor, that is the bone marrow that we're going to inject into the irradiated recipient, and we irradiate that bone marrow very lightly, not to kill the bone marrow cells but to introduce random chromosomal breaks, a very small number of random chromosomal breaks in the donor bone marrow. So, the purpose now of irradiation is quite different from what I said before. Before we wanted to give a heavy dose of radiation to wipe out the recipient bone marrow.
Now we're going to just give a wee bit of radiation to the donor bone marrow. What's the purpose of that? The purpose of that small amount of radiation is to create chromosomal abnormalities.
So, for example, if here are two homologous chromosomes in the donor cells. Since the radiation, the very weak dose of radiation is acting randomly it will create all kinds of abnormalities including, for example, a very specific chromosomal translocation so that what might happen after this is that a whole chunk of this chromosome is translocated over to this chromosome here. This is called a chromosomal translocation.
And now here's the donor, these are donor bone marrow cells. And keep in mind that every donor bone marrow cell that gets a little bit of this radiation will get its own very specific randomly occurring translocation just because radiation is able to break chromosomes and then they will rejoin in unpredictable ways. What that means is that if we take the donor bone marrow and irradiate it very lightly so that we don't kill the cells but we do induce these translocations, one donor cell will have this translocation and another donor cell over here will have a totally different translocation from a different chromosome also induced randomly by these stochastic processes. So the karyotype which is the whole array of chromosomes of a cell, which can be viewed at the metaphase of mitosis when all the chromosomes condense, the karyotype of each of these donor bone marrow cells will be messed up slightly.
And it will have recognizable abnormalities, but they're all different, one after the other. And after we've done this, after we've marked millions of bone marrow cells in the donor with these random low-dose radiations, we can then inject a small number of bone marrow cells into the recipient. And what we can sometimes find on occasion is if we look at the recipient after that recipient has been rescued, i. ., after the donor bone marrow has established itself within the recipient, is that the donor bone marrow is established in the recipient and populates all of these different lineages. And if we're able to look at the karyotype of these different kinds of cells in the recipient organism, we can find that in some mice all of these cells have the same very peculiar translocation. They have either this one or this one or they have yet a third translocation, any one of a whole series of randomly occurring mutations, a very peculiar idiosyncratic unusual translocation induced by the low-dose radiation. They all have it.
The T cells and the B cells and the monocytes and the basophiles, they all have exactly the same translocation.
Obviously, we can't do that experiment with the platelets.
Why? Because they don't have nuclei. And we can't do that with the erythrocytes either, the red blood cells. Why can't we do that? Because in mammals, when the red blood cells are formed, the nuclei are spit out. Our red blood cells don't have nuclei in them anymore. They've become enucleate or, to put it another way, they have been enucleated. That is to say they've been deprived of their nuclei. Why? Because they're post-mitotic.
Obviously, a cell which lacks nuclei is by definition post-mitotic.
And our cells don't really need, red blood cells don't need nuclei.
We know that ancestral organisms, for instance chickens, their red blood cells are nucleated, but our red blood cells are not because they're just not necessary.
How is translocation different from crossing over?
Crossing over occurs between two homologous chromosomes.
So if we have a chromosome here, here's chromosome 13 and here's another chromosome 13, they're both chromosomes 13.
This one came from Ma. This one came from Pa. All right?
Each of us has a pair of homologous chromosomes.
Here's the maternal one. Here's the paternal one.
When we talk about crossing over we're talking about a process of homologous recombination.
And when that happens we have a situation like this.
This chunk is exchanged with this chunk over here.
It's an equal exchange, absolutely equal down to the nucleotide, so that after this flipping has occurred we have two fully intact chromosomes. It's just that both of these chromosomes are fully normal. It's just that there's been a switching, an exchange between the two homologous chromosomes, the two paired chromosomes 13. When we talk about translocation there a chunk of chromosome 15 can go onto chromosome 7 or a chunk of chromosome 2 can go on chromosome 8. It's totally random, it's non-homologous, and it creates aberrant chromosomes.
Neither of these recombined chromosomes is abnormal.
It's just changed it allelic configuration.
So, it's an important distinction. And the fact is you can pick out these translocated chromosomes because one even has specific dyes that can be able to tell you which chromosome this came from and which chromosome this came from. So, there is a profound difference.
Translocations are, invariably, pathologic. When I say pathologic, I mean they're really sick. They're not the proper course of things that happens in a healthy cell. The fact, the very fact that we're able to generate an entire array of cells in the blood indicated, by necessity, that if all these cells have the same chromosomal translocation that they descend from a donor cell that originally was lightly irradiated and happened to receive that translocation. If we never get this array of common translocations in all the cells in a bone marrow recipient then we can't prove this, but the fact is this has been proven time and again over the years. And this indicates to us that this cell which is genetically slightly altered can, therefore, generate all these other cells in the body. Again, keep in mind that the irradiation of the donor marrow is simply to create these chromosomal markings. They're not necessarily good for the organism, but they don't compromise the viability of the cell.
They just reshuffle the chromosomal structure. Now, in principle the levels of each one of these kinds of end-stage cells need to be carefully regulated. And, by the way, let me just note here, you see the T cells have the arrow going back on themselves, as do the B cells. That indicates that they have not become post-mitotic. Remember we talked about these embraces between helper T cells and B cells where they're walking down the allies and they get excited and they start multiplying? The fact that the T cells and the B cells are able to proliferate in response to certain antigenic stimuli implies that they're not post-mitotic. They still have the ability to proliferate, and that retained ability to proliferate like that of hepatocytes is indicated by these arrows that are looping back on themselves. Conversely, these cells are all essentially, as I've said before, post-mitotic. So how do we insure that's there proper concentrations of all of these different cells in the blood? And the fact is the concentrations of many of these cells in the blood are maintained to concentrations of plus or minus 10%. And this is, itself, a stunning testimonial to the successes of human physiology.
We're talking here about a process which is sometimes called homeostasis. Homeostasis means that somehow there is a balance, an equilibrium that is achieved, and that there aren't profound fluctuations so that we always have roughly the equal level of red blood cells, a proper level of lymphocytes in our blood.
And I want to get into the homeostasis which results in the formation of red blood cells, the RBCs, the erythrocytes in the blood. In fact, it happens to be the case that the red blood cells are one of the cell types that could actually vary quite profoundly in response to environment.
To the extent that lymphocytes change, they go up and down, that might be due in response to an infection. So, if we have a serious bacterial infection we might have increased in the lymphocytes in the blood that have been mobilized in order to attack the infecting bacteria. But what about the RBCs? What about the red blood cells? What causes them to change?
Well, if you move from here to Denver, Colorado or you go up skiing in the Rockies, you're going up to ten or twelve thousand feet. And within a matter of three or four days the concentration of your red blood cells increases very substantially. Why? Because obviously the oxygen tension at high altitude is down. And in order that your peripheral tissues become adequately oxygenated, the oxygen carrying capacity of the blood must be increased. And the way that is increased is in part to increase the concentration of red blood cells.
So how does that happen? How is it possible that we can rapidly modulate the concentration of red blood cells?
And the way we can do that is in part through a hormone called erythropoietin, EPO. We're going to talk about erythropoietin at the beginning of the lecture next time, but the homeostasis which maintains the appropriate number of red blood cells in our circulation is dictated in no small part by the levels of EPO that are in their blood. To anticipate some of the things we're going to say next time, when you are in a low-oxygen environment the levels of erythropoietin shoot up.
And when they shoot up they insure that there is shortly thereafter a rapid increase in the level of circulating red blood cells which in turn enables the oxygen coming into your lungs to be transported more efficiently, more effectively into the peripheral tissues.
You've heard about athletes perhaps who are able to dope themselves with erythropoietin. This is a rather devious strategy because it means that if they do so, they inject themselves with a little erythropoietin, the oxygen carrying capacity of their blood is temporarily increased and as a consequence they might be able to run further or jump higher. This, by the way, has its dangers. Because if you're injecting erythropoietin not in response to certain physiologic signals but just because you want to win a marathon or something, you're violating the normal physiologic mechanisms in the body which very carefully control the levels of erythropoietin. And if you inject too much erythropoietin you get in a very bad situation because the bone marrow makes more and more red blood cells. And then what happens? You start clotting up everywhere all over the body, and this isn't good.
In fact, you can die. So this is not a warning against erythropoietin in the way that I warned you against cigarettes.
This is just to tell you these kinds of drugs, or these kinds of growth factors, which they are, are maintained at very precise levels as we'll discuss in more detail next time.
See you on Wednesday.
Free Downloads
Video
- iTunes U (MP4 - 130MB)
- Internet Archive (MP4 - 130MB)
Audio
- iTunes U (MP3 - 12MB)
Free Streaming
Subtitle
- English - US (SRT)