Flash and JavaScript are required for this feature.
Download the video from iTunes U or the Internet Archive.
Topics covered: Molecular Biology 1
Instructors: Prof. Eric Lander
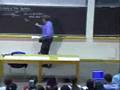
Lecture 10: Molecular Biolo...
Good morning. Yeah. All right. Good.
Something to counteract the rainy days we have here.
All right. Today we're going to make a very important transition.
The transition goes back to this picture.
Of course, what we want to do is understand biological function by taking our two favorite approaches.
Understanding the organism minus an individual gene.
Understanding an organism minus an individual component, and understanding the individual components minus the organism, genetics and biochemistry. And, as we know, the geneticist went off on their route finding mutants, doing mutant hunts, making crosses, making genetic maps, et cetera, didn't understand really what these genes had to do with anything specific in the organism, other than they produced phenotypes when they were mutated.
And the biochemist went off purifying enzymes, working on biochemical pathways, et cetera, et cetera.
We began to see some connection when we talked a bit about the mutants that affect the ability to make arginine and the fact that they could encode different enzymatic steps. And, in particular, I highlighted the work of Archibald Garrett who really, right at the start of the century, recognized that somehow genetic mutations were responsible for somehow affecting the production of enzymes in important biochemical pathways.
So, that was sort of one connection of genetics into protein but still a rather tenuous one. The real next step to connect these two would be to do the biochemistry of genes. So, how would a biochemist approach heredity? A biochemist would grind up the organism, fractionate it in different components, and attempt to find heredity, purify heredity, get like a pure solution of heredity. And that's nuts, right?
The notion that you could purify heredity as a biochemical entity because like how would you know you had it? But, of course, that's exactly what happened. That is what happened, was biochemistry developed sufficiently far that folks were actually able to purify substances, not just that could digest a particular sugar or substances that might, you know, like slide by each other like actinomycins of muscles, but substances that were actually heredity. And that began the real unification of those, and that's the point of today.
And that is the field of molecular biology. And we're going to cover tremendous territory in unifying these two different fields.
OK. So, let's dive right in. The discovery of the transforming principle. It's a wonderfully old-fashioned kind of word. Nobody would use language like this today.
The discovery of the transforming principle. So, this starts, this shaggy dog story starts in about 1928 with the work of Griffiths. Griffiths had no particular interest in DNA or genetics or biochemistry for that matter.
Griffith was interested in bacteria. He wanted to understand bacteria.
And, in particular, he studied pneumococcus bacteria, which could infect and kill mice. And he was very interested in the mechanism by with these pneumococci could kill mice.
Now, it turns out that the pneumococcus bacteria came in two different types. One, the bacteria, when they grew on a Petri plate, produced a glistening, smooth, shiny colony. We'll call them smooth bacteria here.
And these bacteria, in addition to being smooth and glistening, were virulent. That is, if you inject into the mouse, these bacteria would kill the mouse. They are smooth because they have this encapsulating polysaccharide coat around them.
And it's not necessarily the case that that's what makes them virulent, although it actually does have a role in that, but it is the case that they're smooth and they're virulent. So, you inject in a mouse, mouse dies because the mouse is not resistant to these bacteria. By contrast, there were strains of pneumococcus that were rough. They did not have the same kind of a polysaccharide coat, and therefore they had a very rough appearance. They didn't glisten.
And these were non-virulent. If you inject these into the mouse, the mouse immune system was able to fight these particular rough bacteria. OK. So, Griffith did the obvious experiments. So, take some bacteria, we'll take some smooth virulent bacteria, we'll inject into a mouse. And what will happen?
The mouse will die. This is one of the easier assays in the laboratory.
It's the feet up, feet down assay, you have a dead mouse.
OK. Number two. Then take the rough bacteria, inject it into a mouse, what happens?
Sorry? It lives. The mouse lives because these are non virulent. OK. Now, let's just do some simple controls. Let's take the smooth bacteria and autoclave them, heat them up to very high temperature to kill them.
How will we know they're dead? You try plating them out. They don't grow anymore, so they're dead.
So, take heat killed, and you can verify in the lab that they're killed, heat killed smooth, check that they really were heat killed, inject them into the mouse.
And what happens? It lives because, I mean, they're bacteria, right? OK. Last of all we take the utterly harmless rough bacteria, plus the utterly harmless heat-killed smooth bacteria, we inject them into the mouse, and what happens?
It dies. That is a notable result because the rough bacteria alone will not kill this mouse and the smooth bacteria that have been heat-killed will not kill this mouse, but together they killed the mouse.
This is very puzzling. What was even more puzzling was when you autopsied the mouse, you can isolate from that mouse smooth, virulent, live bacteria, but you didn't put any in. Very strange. So, this actually yields live smooth virulent bacteria despite not having put any in there.
Virulent bacteria. Somehow we were able to create smooth and virulent bacteria notwithstanding not having put any in here. So, of course, Griffith then attempted to say, well, what was it that allowed this to happen?
So, he could try putting in dead rough bacteria with dead smooth bacteria. That doesn't do anything. You need to have something alive.
So, you've got to have live rough bacteria. You can then say let me take the dead virulent bacteria and start fractionating it biochemically and asking what fraction of that material from the dead bacteria allows us to recover, to have this property of being able to now produce virulent bacteria that killed the mice?
And do you realize how utterly tedious and painful that experiment is? You take the dead bacteria, you fractionate it into lots of different biochemical fractions.
For each fraction, how do you test whether it has the property?
You've got to shoot up a bunch of mice. This is a very tedious procedure. I mean it is, you know, you can't underestimate how important the assay is, how important it is to come up with easy ways to do things in order to be able to accelerate progress.
Griffith tried hard and roughly began to purify fractions and get information about what the fractions were. But, in fact, this work really never did lead to a clear conclusion.
But it did tell people that there was some material, which got named The Transforming Principle. This is almost like an old alchemical kind of word, a principle being a particular chemical composition of matter, which if you don't know what it is you call it The Living Principle or something like that.
So, what was this transforming principle?
Well, it really took work about 15 years later by Avery, McCarty and MacLeod to sort this out. What Avery, McCarty and MacLeod did was the same experiment basically, except minus the mice.
What they found was you could take the dead bacteria, combine it, the dead virulent bacteria, the dead smooth bacteria, combine it with the live rough bacteria, and by combining it in the right way in a test tube you would be able to plate it out on a Petri plate and see smooth bacteria come out.
Sans mouse. So, they didn't need the mouse.
This dramatically accelerated work because if you're able to just take fractions of the dead bacteria, add it to the live bacteria and look for the presence of some smooth bacteria coming out of it, you would be able to work much more quickly. And they did.
And they began purifying. And they began purifying and they tried to isolate the fraction that contained this new ability to make these bacteria acquire a new property. And they knew that they were transforming the heredity of this bacterium.
They were transforming the traits of this bacterium.
They were, in fact, transmitting heredity.
And they purified and purified and purified. And eventually, testing many, many fractions and making them purer and purer and purer and purer, they found that consistently the fraction that contained heredity was the fraction that contained DNA.
Now, it was a lot more work than that because no fraction is pure.
DNA is in multiple fractions. But, you know, they kept trying to purify it. And it sure looked like the transforming principle. The property of being able to transform was co-purifying along with the DNA fraction.
And you know what the reaction to that was? Well, mostly it was that they must have goofed because all smart right thinking people knew that DNA was an absolutely boring molecule.
Because the interesting molecule at this time was proteins.
Everybody knew there were zillions, there were 20 amino acids, they came in zillions of combinations, they had all sorts of different shapes and properties, and hydrophobic ones, hydrophilic ones that are enzymes. And, clearly, anything as important as heredity was not going to be encoded in some utterly boring structural molecule that was just a long polymer of four virtually identical units. And so, the sort of reaction was this is interesting but there must be some trick, something must be wrong in this experiment, give or take.
Now, why did people think that DNA was so boring?
Now, DNA had been known for a long time, since the 1860s.
Lots of molecules were known, but why was DNA boring and why were proteins kind of exciting? So, for that, we really do have to look some more closely at the structure of DNA.
I want to review the structure of DNA here because we're going to use it a lot. So, DNA has three components, as you undoubtedly know. It has first a sugar, or almost sugar, two prime deoxyribose, two prime deoxyribose, so it's a pentose, or almost the deoxypentose. And its structure, and this is an important structure. In order to be a true sugar, to be ribose you would have a hydroxyl. Deoxyribose just has a hydrogen there. And the way we number these carbons around this five carbon sugar are very important and we'll always talk about them, the one prime, two prime, three prime, four prime and five prime carbons of this deoxyribose.
And you'll notice that it's the two prime carbon that is dioxi.
So, that's the sugar. The next important component as we build up DNA is the base, OK? The base is put here.
Now, I'm going to start simplifying our sugar.
Base. So, there are four kinds of bases that can go here.
And they are adenine, guanine, thymine, cytosine.
So, that's the second important part of building up DNA.
The third important part in building up DNA is to make the monomers that are used to produce DNA, we need to put on a triphosphate. And he we go. We'll take our sugar here, our base over here, and then off this carbon we have our phosphate. And we have a triphosphate.
There we go. So, this is the monomer that is used to build up DNA.
This guy here is called a nucleoside, note the S.
This guy here with the triphosphate on it is called a nucleotide.
It's not usually written with such a big capital letter, but nonetheless I point this out. And, obviously, what is this triphosphate going to do for us? It's going to provide the energy to allow us to make DNA polymer chains. We're going to do a dehydration synthesis where we break two of those phosphates off and use it for the energy to be able to catalyze DNA chains to be made.
OK. Now, when you combine nucleotides into a DNA strand you do so to create a sugar phosphate backbone. And you'll see for many molecules, I don't care that you know their structures terribly well. But for the basic structure of DNA, including its sugar phosphate backbone, it's going to be important for all that we talk about. So, what happens is we have a chain of DNA growing like this, and we have our OH here.
We have our base here. And which carbon is this?
Five prime, that's right. OK. Which carbon is this? This one.
Great. That's the three prime, two prime, one prime.
Great. OK. To this three prime carbon we add this triphosphate breaking off two phosphates there. The diphosphate gets broken off, the pyrophosphate. And we get a single phosphate linkage to the next subunit of the chain. So, here we go phosphate, sugar, phosphate, sugar. And if we ignore these bases, which, you know, who cares about the bases anyway, what we have is just phosphate, sugar, phosphate, sugar, phosphate, sugar, phosphate, sugar. OK? So, it's a very simple structure.
There's nothing hard to remember about this. And the phosphate is always attached to the three prime carbon of the preceding sugar and to the five prime carbon of the next sugar.
OK? So, we often speak of chains of DNA growing from the five prime end to the three prime end. And that confuses non-molecular biologists to no end. What are we talking about, five prime ends and three prime ends? This is what we're talking about.
But the additions are catalyzed onto the three prime carbon of that sugar. It grows at it three prime end. So, you have sugar, phosphate, sugar, phosphate, sugar, phosphate. So, that's it.
We're all done. Well, there are the bases, I guess, too, right? So, we'll mention these bases.
The bases are, they come in two types. There are purines.
Adenine and guanine are purines. And there's a six member rings with a five member ring. And there are two bases that are called pyrimidines, they are smaller, the thymine and the cytosine. And there are six member rings. And they have some carbon, some nitrogen, some oxygen and some hydrogen.
But, you've got to admit that compared to proteins, this is pretty boring. It's just one long sugar phosphate chain. And two purines, slightly bigger things, two pyrimidines, slightly smaller things. Very similar structures for these two. I haven't even bothered to focus on the difference. And as compared to the richness of proteins, there's just no way anything interesting could happen with this. That was certainly the thinking at the time.
You have to understand how important prior ideas, prior prejudice is to science. People look at it and say this must be some structural molecule. It is scaffolding.
It's like the studs in the wall of the house you're building or something like that. Not too interesting.
So, what happens? Well, you know, it takes time to sort things out. People come back to this problem. Any thoughts?
I mean I've given you one reason why this did not make a huge impact, because it was, you know, DNA was kind of a boring molecule and people weren't really sure this was right. It could be an artifact, right? Maybe some important protein had come along for the ride with the DNA faction, right? What's another reason why people might not have paid tremendous attention to this result?
Sorry? It was just bacteria. Anything else? Couldn't imagine, right, how this DNA could encode the enzyme. Anything else? Date.
It's in the middle of the Second World War. Maybe people had more important things to do, right? So, this is right in the middle of the Second World War, too. It's just worth noting that these guys are working in the middle of New York City at the Rockefeller Institute and it's in the middle of the Second World War.
Anyway, war is over and some work continues on this.
And the work takes a somewhat different attack.
Instead of working bacteria it now is, there's work here on certain bacterial viruses. So, instead, see, bacteria get their own here. Instead of using bacteria to infect mice, Hershey and Chase, and others at the time, used viruses to infect bacteria. So, here the bacterium is the victim. And people had found and had studied these amazingly interesting really tiny things that could affect a bacterium and kill it. These particles that had these funny shapes were called bacteriophage. What does phage mean? To eat.
Bacteria eaters. Bacteriophages were these little viruses. They were incredibly tiny. You could filter them through very small filters. And, yet, when you added them to bacteria they would kill the bacteria.
These were very simple things. I'm reluctant to call them creatures. Are they alive? This is a favorite question people would like to debate. They say are viruses alive?
And the answer is who cares? I mean it depends on what you want to define alive to mean. To me it's not alive in that it cannot replicate on its own without a host, so I won't call it alive.
But, anyway, I'll refer to them loosely as these creatures that eat bacteria. They were very simple. And all they really had in them was some DNA in their capsid, this capsid up here, and some protein. But they could attach to a bacterium and after a certain amount of time cause the bacteria to burst open and produce lots of daughter-phage, lots of daughter bacteriophage. It could replicate within this bacteria.
So, somehow this, while I might not want to call it alive, certainly can reproduce itself, or at least with the help of a bacterium can reproduce itself. When people first discovered this bacteriophage, what do you think they wanted to do with them? Sorry? Yeah. Where? In humans.
The first thought about what to do with bacteriophage were a whole bunch of interesting Russians who wanted to make up large quantities of bacteriophage and have people drink them.
So, they would kill all their bacteria. And this was the early ideas for antibiotics. It didn't quite pan out that way.
But, you know, people have all these very exciting ideas of, wow, I've got something to kill bacteria, let's pour it down a patient and see if that does something good for them.
You know, that's why there are institutional review boards, too, to make sure that you can't just do that right off the bat.
Somebody else has got to think about it also.
It turns out not to be a great way to kill patients, to kill bacteria, sorry. No, it doesn't actually kill patients, but it doesn't also kill the bacteria so well in human beings.
So, anyway. So, the question was how is it that these viruses kill the bacteria? Somehow they inject something into the bacteria, something causes something to happen, which causes virus particles to be made. I don't put too fine a point on it because that's all you could really say at that point, something goes in and something comes out.
So, what goes in? How could we tell what goes in?
Yup. By seeing what's left out. How could we see what's left out?
Just being really practical, how are we going to tell?
Visually look, but that turns out to be terribly hard thing to do.
You've got to have really good eyes to be able to say the protein is still there but not the DNA, or the DNA.
Because the thought was if this thing is injecting its DNA then the DNA must be carrying the instructions to make phage, and this would be hereditary material. So, what we want to show is that the protein stays out and the DNA goes in. But how is that going to, how do you do that practically?
Radioactive labeling turns out to be the best way to do that.
If we could label radioactively the DNA with one label and the protein with a different label, we could see which radioactive isotope goes into the bacteria. Any candidates for an element that we could use to label DNA that won't be in protein?
Sorry? I'm sorry? Oh, who had one? Uranium.
Somebody's thinking World War II here, right, there would be some spare uranium around. The problem with that is that DNA does not actually have uranium in it, and so when you put uranium in it wouldn't still be DNA. We would like to label it with an element that's actually in DNA. So the only difference is that it's a radioisotope. Phosphorus. Phosphorus.
Well, there's obviously phosphorus in that sugar phosphate backbone.
Is there phosphorus in a typical amino acid? Any of the 20 amino acids? No phosphorus. Great. So, we could use a phosphorus isotope. We could P32 label the DNA.
But how do make live bacteriophages that are labeled with radioactive phosphorus? I mean what kind of fancy chemistry do you need to do that? Yes? Perfect if you grow the bacteria in radioactively, in medium. If you grow the virus and the bacteria in medium that has radioactive phosphate, the bacteria and the virus take care of it for you.
The phosphate is automatically incorporated. So, you don't have to do any chemistry. You just feed phosphate, radioactive phosphate into the medium. And the phage that are produced will be radioactively labeled.
Purify them and use them in your experiment. Similarly, what are we going to label our proteins with?
Carbon? No. Hydrogen? No. Oxygen? Nitrogen? No, because the bases have nitrogen. Sulfa. We've only got sulfa.
Where is sulfa going to be? So, for example, cysteines with thiamines, right, we've got sulfa. Here's S35.
So, we can take bacteria and we could, we can take phage, and by growing them in the presence of radioactive DNA, no, radioactive phosphorus, P32 and growing them in the presence of radioactive sulfa, S35, we are about to produce bacteriophage that are labeled. OK? So, P32, S35. Now we infect bacteria with them. Let me take a big tube here.
I'm going to add bacteria. I've got the phage here. The phage particles are attached to the bacteria and they are going to inject whatever they inject. Now what do we have to do? We've got to knock off the bacteriophage particles from the bacteria.
I want to knock them off and see what is staying with the viral particles and what goes into the bacteria. So, how do I get in there with tweezers and separate off, peel off each virus from the bacteria?
Washing turns out not to be strong enough. You've got to previal to get these things off, so you really need some incredibly strong agitation. So, specialized devices were used to create intense agitation. What specialized devices you're aware of that do that? Blenders. Kitchen blenders.
The Waring blender turns out to be the perfect laboratory device for this experiment. And this is actually known as the Waring Blender Experiment. You take the bacteria with the phage attached to it, you let them attach and do whatever they're going to do, inject their DNA, as we know turns out is the right answer. And then you press puree and then vrrrr, and the viral particles fall off. So, it's important to know how things really happen. So then what happens is the bacteria are separated from these particles.
And it turns out these particles are, the viral particles are much lighter, much less dense than the bacteria. So, how do we separate them?
Centrifuge them. We centrifuge them.
The bacterial particles are there up in the supernatant turn out to be our phage capsids. And now what do we do?
We take this stuff, we measure the radioactivity in the supernatant, that is the material that stays above, and we measure the radioactivity in the pellet. And what do we end up seeing?
Where does most of the P32, what shows up in the pellet?
Mostly P32 shows up in the pellet. Is there no S35 in the pellet? You know, in the textbook story, of course, there's no S35 because they want it to be nice and clean. But in reality there's going to be some S35. But it was, you know, less than 1% of the S35 ends up in the pellet. Most of the S35 stays up here in the supernatant. Does all of the phosphorus go in?
No, of course not. Some of the viruses didn't even attach and not everything goes in. So, there's still radioactive phosphate up in the supernatant. But the striking this is that the pellet primarily has gotten the radioactive phosphorus, not the radioactive sulfa, and therefore we can conclude that what? Well, more DNA went in than protein. Are we therefore entitled to include that DNA is the hereditary material? Why?
Well, I mean suppose that 1% sulfate is tracking one minor protein that is the secret. You can't, it's very hard to rule out that there's no contaminants traveling along with the DNA.
And if you really truly disbelieve DNA, you could be churlish and say, well, I just don't believe that you've so purified it that you can completely rule out that some minor protein component is really conferring heredity. In fact, when you really look closely, Avery, McCarty and McLeod's biochemistry, I believe, was purer than the purity of this experiment.
But by this point thinking had begun to shift toward DNA being a reasonable hereditary molecule. In addition, it was the second line of proof, different from the pneumococcus, using a different system, both pointing to the same answer. And the intellectual tide shifted to recognizing that this probably was right, and the reason these experiments were pointing to DNA was DNA had to be the right answer. But, of course, how was it the right answer? What was it about DNA that could confer these properties? This was still unclear in 1953, but not for that long. It became clarified relatively soon thereafter.
And, of course, it became clarified with the understanding of DNA structure, the double helix.
Nobody here has not heard of the double helix. Probably there's nobody, no grownup who doesn't know about the double helix and all that, but nonetheless I want to stop and think a little bit also, I'll say on a personal note, this is the first year I've taught this class after, the first time I've taught this class when Crick and Watson have not both been alive.
Some of you may know that Frances Crick dies just this past summer.
Which was very sad. He was an incredible person and, you know, as I've said, Mendel was one of my heroes.
Francis Crick was also one of my heroes. He was just an extraordinary person. But Jim Watson is still alive and kicking and still quite active. And so, in any case, you're not far removed. So, I tell you a little bit about this stuff as history, but this history I'm telling you about, these people are, for the most part, Francis' passing notwithstanding, alive and kicking. Jim Watson is still quite alive.
Actually, McCarty is still alive. It's really, anyway.
So, 1953, just a year later, Jim Watson and Francis Crick are working in England. Watson is a student for Indiana, a former ornithologist, had his interest in ornithology originally, and then studies more biology and came to England because he wanted to study the gene. Francis Crick, a physicist who worked in the admiralty during World War II.
And, of course, what they did was on the basis of an awful lot of modeling and getting to see experimental x-ray diffraction pictures of Roselyn Franklin from London who made a model.
And the model is this beautiful, and I haven't drawn it to its proper proportions, but this beautiful double helical structure.
Five prime, one chain of DNA running in one direction, five prime to three prime. An anti-parallel chain of DNA going in this direction, five prime to three prime.
It was a beautiful structure. Jim Watson has written a whole book about the discovery of the double helix structure, and we are only 51 years past that. It was, anybody who hasn't read The Double Helix, this book, really should. It's one of the treat books of science literature, and actually is on many people's lists of some of the great books of the 20th century.
It's a wonderful competitive story of Crick and Watson racing against Linus Pauling. It's, you know, someone came along and had lunch in Cambridge with Crick and Watson.
And they came away and said, this was before they discovered the structure, about a year or so before, and said these guys are idiots.
They can't even memorize the structure of A and T and C and G, and they're trying to find, you know, the structure of DNA.
These guys are never going to get anywhere. So, this person, who we'll come back to in a moment, was wrong about this particular point. Because what Crick and Watson did was they played around with the models, and what they ended up noticing was a couple of things. First off, from Rosalyn Franklin's pictures, that this was helical. The x-ray to fraction pictures could tell you at a glance that the structure was helical.
They saw that. They then tried to make helices. Now, other people, Linus Pauling knew something that DNA probably had to be helical, and somehow he just got it totally wrong.
He made just a nutty model of DNA. Linus Pauling, the smartest chemist of the century made a crazy model of DNA where he took the sugar phosphate backbones and put all the sugar phosphate backbones in the middle, and had three of them. He had a triple helical model with sugar phosphates in the middle. And what can you tell me about the charge on these sugar phosphate backbones? Very negative.
You're going to stick a whole bunch of negative charges near each other in the middle? No way. Anybody could have known.
This was a bush-league mistake, so Crick and Watson said phew, Pauling has got it wrong. They put together this model.
And the key to the model was the recognition of base pairing, the recognition of base paring. That if I take a thiamine here and I take an adenine here. That these two groups would be pointing at each other in such a way as to make two hydrogen bonds with a certain characteristic distance. And, not just that, but cytosine and guanine could also be fit into that same distance.
And they would have three hydrogen bonds.
And here NH, H, doo, doo, doo, doo, doo, doo, doo, three hydrogen bonds. And they would fit the, what do I got? Oops. Thank you. Good point.
That's the problem. Yup. Well, it's a little messy but anyway. The business end here is three hydrogen bonds and two hydrogen bonds, and they both fit into the same distance perfectly. So, this double helix here could have either As and Ts or Gs and Cs or Cs and Gs or Ts and As.
And they would all fit perfectly with each other.
Now, there was an old observation, not that old, there was an observation floating around at the time that said when you analyze the amount of As and Ts in DNA, you always found out that the amount of A tended to be very close to the amount of T. The amount of C tended to be very close to the amount of G.
Although, these amounts could be different. This was due to a biochemist called Chargaff. And they're called, this was called Chargaff's Rule or Chargaff's Law or Chargaff's Observation.
Chargaff noted that the percentage of these amounts tended to be equal but didn't know what to make of it. This perfectly explained that.
It was very good. Remember I said somebody came through Cambridge and said these guys were turkeys, Crick and Watson were turkeys because they couldn't even remember the structure and all that? This was a very distinguished chemist who said this about Crick and Watson. It was Chargaff.
Chargaff came through and said these guys are turkeys.
But it was Chargaff's Rule that Chargaff had missed the importance of. He was quite bitter about this through much of his life.
And there's a very wonderful biting quote that Chargaff says when Crick and Watson become famous for the DNA double helix.
Let's see if I can get it right. He says that such pigmies should cast such giant shadows, referring to Crick and Watson, that such pigmies should cast such giant shadows only shows how late in the day it is. Anyway, he was not happy.
So, all right. Now, this was a big deal thing.
Crick and Watson knew this was very important. They raced to publish a paper about this. They sent it off to Nature.
It's a gem of a paper. It's a page roughly in text.
It's very short, very clear, has this beautiful picture drawn from Francis Crick's wife, Odile. And it is just a charming paper. They know that they've cracked the secret of life. Why do they know they've cracked the secret of life? Because the most important thing about this model here is not its structure per se.
But that it explains how it is that a DNA molecule can be replicated, that somehow all it takes is for those two strands to come apart, who knows how, and that when they come apart each can serve as a template for the other because since As always match Ts and Cs always match Gs, each strand has enough information for the other.
That's how replication happens. You have two strands, each of which has sufficient information to encode the other.
They somehow come apart. They each serve as a template for the other. And that's that. That is the secret of life, how life replicates itself. Not just that. We've explained replication. What about mutation. What's mutation? It sometimes gets it wrong. It sometimes screws up. So, for one little biochemical model we've explained replication and mutation. That's pretty good.
Now, the thing is, in writing this paper and getting this off to the Journal, this was not an easy thing to get done quickly.
You couldn't, you just didn't have time to explain all these details.
They wanted to stake their claim about this, so they wrote up the structure. And instead of going through a long about how this explains replication and dah, dah, dah, dah, dah, dah, dah, dah, there's one sentence in the paper, the last sentence of the paper in which they just say it has not escaped our notice that this model explains replication and everything else. Basically the last sentence says, oh, and by the way, it has not escaped our notice that this explains the secret of life. Although, it didn't say it like that.
It's the coyest sentence in the scientific literature.
It's really just an amazing sentence there.
And then they come back a couple minutes later and write a paper explaining what they mean and all that, but it's just a great sentence.
So, you will hear molecular biologists make reference, use in their speech the phrase, it has not escaped our notice that.
And it's always a homage back to this particular sentence in this paper of Crick and Watson. OK, now, last thing. Yes?
Jim Watson was 25 and Francis was 35 when he did this.
Yes. He was a 25-year-old kid when he did this. That's right.
Pretty amazing stuff. So, last point I want to touch on, and I'm not sure I'll get to, get all the way there, but this model, this model here of DNA coming apart and each strand serving as a template for the other strand is called Semi-Conservative Replication. That is one strand is used as the template for the other strand, so there's an old strand and a new strand that's made. In theory, you could imagine that DNA replication occurred not like this.
But instead somehow, I can't imagine how, but you could imagine, and people were willing to imagine it, that the old strands stayed together but somehow became a template for making a new double helix without actually using them. This model of the strands actually serving as a template would predict that each new DNA double helix was, in fact, composed of one old and one new strand.
If you could prove that then you'd have real confirmation of this Crick Watson model, semi-conservative replication. And so, a young student, two young students, Matt Meselson, who's still working and is down the road at Harvard and a wonderful person, and Frank Stahl, who's still working in Oregon, proved that the new DNAs that were made after each generation were, in fact, composed of one old strand and one new strand.
How could you possibly do that? Sorry? Radioactive labeling. But how do you radioactively label it so that you can see that it's, that you've got a double helix that's half one and half the other?
Old ones labeled with, well, with one isotope. It actually turns out to be nitrogen. The new one with a new isotope.
Say N14. You can do heavy nitrogen and ordinary nitrogen.
And if what you could do is grow up your DNA, when you first grow it in normal nitrogen, then you shift to N15, heavy nitrogen, you could make DNA molecules that were half old, half new, and therefore half labeled with normal nitrogen, half labeled with heavy nitrogen. And how would I prove that these DNA molecules were a 50/50 hybrid? What would be the property that I would be able to test? Well, radioactivity turns out to be really hard to weight density. It turns out density, if I could just measure the density of the DNA, I would show that if the semi-conservative model is true, the molecules will now have intermediate density between all heavy and all light nitrogen.
They had to work out a centrifugation technique so sensitive, a salt gradient centrifugation where you could put DNA on it. You had a really fine salt gradient spun in a centrifuge.
And depending on where the DNA migrated, you could measure the density of the DNA. And they were able to show that, in fact, newly replicated DNA strands had this intermediate density that would be expected from the semi-conservative model.
And so, in fact, by that point the semi-conservative model, I think, is well established. In some sense you would say the beauty of the double helix was almost one of these very rare scientific results where when you look at it you say it can't possibly be wrong. It explains too much.
It's too beautiful. But, as we've discussed before, that's not enough. You need some proofs that it's real.
And this Meselson-Stahl experiment provided a real confirmation of that.
Onto next time.
Free Downloads
Video
- iTunes U (MP4 - 165MB)
- Internet Archive (MP4 - 165MB)
Audio
- iTunes U (MP3 - 12MB)
Free Streaming
Subtitle
- English - US (SRT)