Flash and JavaScript are required for this feature.
Download the video from iTunes U or the Internet Archive.
Description: This is the first of four lectures on Thermodynamics.
Instructor: Mehran Kardar
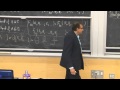
Lecture 1: Thermodynamics P...
The following content is provided under a Creative Commons license. Your support will help MIT OpenCourseWare continue to offer high quality educational resources for free. To make a donation, or view additional materials from hundreds of MIT courses, visit MIT OpenCourseWare at ocw.mit.edu.
PROFESSOR: This is 8.333 Statistical Mechanics. And I'll start by telling you a little bit about the syllabus before going through the structure of the course. So what I have written here is a, kind of, rough definition of statistical mechanics from my perspective. And the syllabus is a guide to how we are going to approach this object. So let's take a look at the syllabus over there.
So the first thing that you're going to focus on is, what is it that you're trying to describe? And these are the equilibrium properties that are described best through what I've been doing section one, which has to do with thermodynamics. You'll start today with thermodynamics. Basically, it's a phenomenological approach, so you essentially look at something, as kind of a black box, without knowing what the ingredients are, and try to give some kind of description of how it's function and properties change.
And these can be captured, for the case of thermal properties of matter through the laws of thermodynamics, which we will set out in this first section, which will roughly take us the first four lectures of the course.
Then I said that statistical mechanics is a probabilistic approach. So we need to establish what the language of probability is. And that can be the topic for the second two and half lectures of the course. It is something that is less physics-y, but since the topic itself has to deal with probabilities, it is very important, from my perspective, to set out the language and the properties of systems that are described probabilistically.
Separately, we'll devote a couple of lectures to do so. And in particular, it is very important that the laws of probability, kind of, simplify when you're dealing with a large number of variables, as captured, for example, by what we call the central limit theorem.
So you can see that the third element of this course, which is the law of large numbers, is inherent also to what simplification you will see in the section on probability. And feeds back very much into how statistical mechanics is developed.
But then we said large number of degrees of freedom. So what are these degrees of freedom? Well, this is now taking a different perspective. For us in thermodynamics, when you look at the system as a black box, and try to develop laws based on observations, we say that well, from the perspective of physics, we know that this box contains atoms and molecules.
And these atoms and molecules are following very specific laws, either from Newtonian mechanics or quantum mechanics. And so if we know everything about how atoms and molecules behave, then we should be able to derive how large collections of them behave. And get the laws of thermodynamics as a consequence of these microscopic degrees of freedom and their dynamics.
And so that's what we will discuss in the third part of the course that is devoted to kinetic theory. We will see that even at that stage, it is beneficial to, rather than follow individual particles in a system, to adopt a probabilistic approach, and think about densities, and how those densities evolve according to Liouville's Theorem.
And what we will try to also establish is a very distinct difference that exists between thermodynamics, and where things are irreversible and going one direction, and Newtonian, or quantum mechanics, where things are reversible in time. And we'll see that really it's a matter of adapting the right perspective in order to see that these two ways of looking at the same system are not in contradiction.
So having established these elements, we will then finally be in the place where we can discuss statistical mechanics in terms of some postulates about how probabilities behave for systems that are in equilibrium. And how based on those postulates, we can then derive all the laws of thermodynamics and all the properties of thermodynamics systems. That they're ordained while observations and phenomenological theories before.
Now initially, in section four, we will do that in the context of classical systems-- description of particles following classical laws of motion. And, again, as a first simplification, we will typically deal with non-interacting systems, such as ideal gas. And make sure that we understand the properties of this important fundamental system from all possible perspectives.
Then in section five, we will go on to more realistic systems where there are interactions among these particles. And there are two ways to then deal with interactions. You can either go by the way of perturbation theory. We can start with ideal system and add a little bit of interaction, and see how that changes things. And we develop some elements of graphical perturbation theories in this context.
Or, you can take another perspective, and say that because of the presence of interactions, the system really adopts a totally different type of behavior. And there's a perspective known as mean field theory that allows you to do that. Then see how the same system can be present in different phases of matter, such as liquids and gas, and how this mean field type of prescription allows you to discuss the transitions between the different types of behavior.
Eventually, you will go on, towards the last quarter of the course, rather than the classical description of matter to a quantum description of the microscopic degrees of freedom. And we will see how the differences and similarities between quantum statistical mechanics, classical statistical mechanics, emerge. And just, historically, of course, radius macroscopic properties of the matter, the black body laws, or heat capacities, have been very important in showing the limitations of classical description of matter, and the need to have something else, such as the quantum description.
We will not spend too much time, more than three lectures, on the sort of principles of quantum statistical mechanics. The place where quantum statistical mechanics shows its power is in dealing with identical particles, which classically, really are kind of not a very well-defined concept, but quantum-mechanically, they are very precisely defined, what identical particles mean. And there are two classes-- fermions and bosons-- and how even if there's no interaction between them, quantum statistics leads to unusual behavior for quantum systems of identical particles, very distinct for fermions and for bosons.
So that's a rough syllables of how the course will be arranged over the next 25, 26 lectures. Any questions about what we're going to cover? OK, then let's go here.
So I will be teaching the course. My research is in condensed matter theory and statistical physics. So this is a subject that I like very much. And I hope to impart some of that love of statistical physics to you. And why it is an interesting topic.
Lectures and recitations will be conducted in this room, Monday, Wednesday, Friday. And you ask, well, what does it mean that both lectures and recitations are here? Well, for that you will have to consult the timetable. And that's probably the most important part of this web page. And it tells you, for example, that the first five events for the course are all going to be lectures Monday, Wednesday, Friday of next week.
And the first recitation will come up on Monday, September the 16th. And the reason for that is that the first problem set will be due on the 18th. And I will arrange for you to have six recitations on the six events that are before the due dates of those problem sets.
Also indicated here is the due dates, naturally, of the problem sets. And although I had indicated that this will be handed out tomorrow, the first problem set is already available on the web, so you can start going to take a look at that. And eventually, also on the web page, will be posted the solutions. And the first one will be posted here. Of course, it's not available yet. Surprisingly.
Once the due date has passed on the date that is indicated, the solutions will be posted. Also indicated here is that there will be various tests. The first test will fall on October 2. And another time and recitations will take place are prior to the tests. So there is actually three tests, and there will be three citations that will take place before that.
And, ultimately, at the end, there will be a final exam. It's date I don't know yet. So I just randomly put it on the Monday of the week where the final exams will be held. And once the actual date is announced by the registrar, I will make sure that I put the correct date and place in this place.
OK, so that's the arrangement of the various lectures and recitations. In addition to me, the teaching staff consists of Max [? Imachaov ?] and Anton Goloborodko. Anton is here, sitting at that extreme corner. Max, I believe, is now in Paris. OK, both of them work on biological systems that use a lot of statistical physics content to them. So maybe they will tell you some interesting problems related to that in the recitations.
At this point in time, they have both set their office hours to be Thursday, 3:00 to 5:00, in their lab, which is close to where the medical facilities are. If you find that inconvenient, you could potentially change that, or you could get in touch with either the TAs, or myself, when you want to have specific time to meet us. Otherwise, I have indicated my own availability to be the half hours typically after lectures on Monday, Wednesdays, and Fridays.
One other set of important things to note is how the course is organized. So I already mentioned to you what the syllabus of the course is. I indicated where and when the lectures and recitations are going to take place. This is the web page that I have been surfing through. And all of the material will be posted through the web page, so that's where you have to go for problem sets, solutions, et cetera.
Also, grades. And, in particular, I have my own system of posting the grades, for which I need a pseudonym from each one of you. So if you could all go through this checking online, indicate your name, your email address, and choose a pseudonym, which I emphasize has to be different from your real name. And if it is your real name, I have to randomly come up with something like "forgot to put pseudonym" or something. I cannot have your real name followed by the grades. OK?
I'll discuss anonymous comments, et cetera, later on. As you will see, I will hand out, through the web page, extensive lecture notes covering all of the material that I talk about. So in principle, you don't need any textbooks. You can refer to the notes and what you write in the lectures. But certainly, I-- some people like to have a book sitting on their bookshelf. So I have indicated a set of books that you can put on your bookshelf. And hopefully consult for various topics at different stages. And I will, through the problem sets, indicate what are good useful chapters or parts of these books to take a look at.
Now how is the grade for the course constructed? An important part of it is through this six problem sets that we mentioned. So each one of them will count for 5%, for a total of 30% going towards the contribution of the problem sets. I have no problem with you forming study groups, as long as each person, at the end, writes their own solution. And I know that if you look at around sufficiently, you can find solutions from previous years, et cetera, but you will really be cheating yourself. And I really bring your attention to the code of honor that is part of the MIT integrity handbook.
We have indicated, through the schedule page, the timeline for this six problem sets are due. They are typically due at 5:00 PM on the date that is indicated on that page, and also on the problem set. And the physics department will set up a Dropbox, so you can put the problem set there, or you can bring it to the lecture on the date that it is due. That's also acceptable.
There is a grey area of about a day or so sometime, between when the problem set is due and when the solutions are posted. If problem sets are handed in during that gray area, they will count towards final 50%, rather than full towards the grade. Unless you sort of write to me a good excuse that I can give you an extension.
Now every now and then, people encounter difficulties, some particular week you are overwhelmed, or whatever, and you can't do the particular problem set, and they ask me for an excuse of some form. And rather than doing that, I have the following metric. That is, each one of these problem sets you will find that there's a set of problems that are indicated as optional.
You can do those problems. And they will be graded like all the other problems. And in case, at some later time, you didn't hand in some problem set, or you miss half of the problem set, et cetera, what you did on these optional problems can be used to make up your grade, pushing it, eventually, up to the 30% mark.
If you don't do any of the optional problems, you just do the required problem, you will correctly you will reach the 30% mark. If you do every single problem, including optional ones, you will not get more than 30%, so the 30% is upper-bound. So there's that.
Then you have the three tests that will be taking place during the lecture time, 2:30 to 4:00, here. Each one of them will count 15%, so that's another 45%. And the remaining 25% will be the final exam that will be scheduled in the finals week. So basically, that's the way that the grades are made up.
And the usual definition of what grades mean-- typically, we have been quite generous. I have to also indicate that things will not be graded on a curve. So that's a MIT policy. And there are some links here to places that you can go to if you encounter difficulties during the semester. So any questions about the organization of the course?
OK, so let's see what else we have. OK, course outline and schedule, we already discussed. They're likely to be something that are unexpected, or some things that have to be changed. Every now and then there is going to be a hurricane almost with probability, close to one. We will have a hurricane sometime during the next month or so.
We may have to postpone a particular lectures accordingly. And then the information about that will be posted here. Currently, the only announcement is what I had indicated to you before. Please check in online indicating that you are taking this course, and what your pseudonym is going to be. OK?
I give you also the option, I would certainly welcome any questions that you may have for me here. Please feel free to interrupt. But sometimes people, later on, encounter questions. And for whatever reason, it may be question related to the material of the course. It may be related to when various things are due, or it may be there is some wrong notation in the problem set or whatever, you can certainly anonymously send this information to me. And I will try to respond. And anonymous responses will be posted and displayed web page here. Currently there is none, of course.
And, finally, something that-- OK, so there's a web page where the problems will be posted. And I want to emphasize that the web page where the solutions are posted, you may see that you cannot get to it. And the reason would be that you don't have an MIT certificate. So MIT certificates are necessary to reach the solution page.
And also they are necessary to reach the page that is devoted to tests. And actually there is something about the way that I do these three in-class tests that is polarizing. And some people very much dislike it. But that's the way it is, so let me tell you how it's going to be conducted.
So you will have this one and a half hour [INAUDIBLE] test. And I can tell you, that the problems from the test will be out of this collection that I already posted here. So there is a collection of problems that is posted on this page. And furthermore, the solutions are posted. So there's a version of this that is with solution. So the problems will be taken from this, as well as the problem sets that you have already encountered-- [INAUDIBLE] solution is posted.
So if you are familiar with this material, it should be no problem. And that's the way the first three tests this will go. The final will be, essentially, a collection of new problems that are variants of things that you've seen, but will not be identical to those.
OK, so where is my cursor? And finally, as I indicated, the grades will be posted according to your pseudonym. So as time goes on, this table will be completed. And the only other thing to note is that there's actually going to be lecture notes for the various materials, starting from the first lecture, that will be devoted to thermodynamics. Any questions? OK.
So let me copy that first sentence, and we will go on and talk about thermodynamics.
The phenomenological description of equilibrium properties of microscopic systems. And get rid of this.
One thing that I should have emphasized when I was doing the syllabus is that I expect that most of you have seen thermodynamics, have done in a certain amount of statistical mechanics, et cetera. So the idea here is really to bring the diversity of backgrounds that you have, for our graduate students, and also I know that our students from other departments, more or less in line with each other.
So a lot of these things I expect to be, kind of, review materials, or things that you have seen. That's one reason that we kind of go through them rapidly. And hopefully, however, there will be some kind of logical systematic way of thinking about the entirety of them that would be useful to you.
And in particular, thermodynamics, you say, is an old subject, and if you're ultimately going to derive the laws of thermodynamics from some more precise microscopic description, why should we go through this exercise? The reason is that there is really a beautiful example of how you can look at the system as a black box, and gradually, based on observation, build a consistent mathematical framework to describe its properties.
And it is useful in various branches of science and physics. And kind of a more 20th century example that I can think of is, Landau's approach to superconductivity and superfluidity, where without knowing the microscopic origin of that, based on kind of phenomenology, you could write down very precise description of the kinds of things that superconductors and superfluids could manifest.
So let's sort of put yourselves, put ourselves, in the perspective of how this science of thermodynamics was developed. And this is at the time where Newtonian mechanics had shown its power. It can describe orbits of things going around the sun, and all kinds of other things. But that description, clearly, does not apply to very simple things like how you heat up a pan of water.
So there's some elements, including thermal properties, that are missing from that description. And you would like to complete that theory, or develop a theory, that is able to describe also heat and thermal properties. So how do you go about that, given that your perspective is the Newtonian prescription?
So first thing to sort of a, kind of, parse among all of these elements, is system. So when describing Newtonian mechanics, you sort of idealize, certainly you realize that Newtonian mechanics does not describe things that we see in everyday world. You kind of think about point particle and how a point particle would move in free space. And so let's try to do a similar thing for our kinds of systems.
And the thing that is causing us some difficulty is this issue of heat. And so what you can do is you can potentially isolate your system thermally by, what I would call, adiabatic laws. Basically say that there's these things, such as heat, that goes into the system that causes difficulty for me. So let's imagine in the same that I'm thinking of the point particle, that whatever I have is isolated from the rest of the universe, in some kind of box that does not allow heat transfer.
This is to be opposed with walls that we would like to eventually look at, which do allow heat transfer. Let me choose a different color. Let's say green. So ultimately, I want to allow the exchange of whatever this heat is in thermal properties to go and take place with my system. OK? Now, this is basically isolation.
The next element is to wait for your system to come to equilibrium. be You realize that when you, for example, start with something that is like this, you change one of the walls to allow heat to go into it. Then the system undergoes some changes. Properties that you're measuring are not well-defined over some period where these changes taking place, but if you wait sufficiently, then they relax to some new values. And then you can start making measurements. So this is when properties don't change.
And the key here is observation time. This is part of the phenomenology, because it is not precise. I can't tell you how long you have to wait. It depends on the system under consideration. And some systems come to equilibrium easily, some take a long time.
So what are the properties that you can measure? Once things have settled down and no longer change with time, you can start to measure various properties. The ones that are very easy for you to identify are things that are associated with mechanical work, or mechanical properties.
And, for example, if you have a box that contains a gas, you can immediately see well, what's the volume of the gas? You can calculate what pressure it is exerting on its environment. So this is for a gas. You could have, for example, a wire. If you have a wire, you could calculate, rather than its volume, its length and the force with which you are pulling it.
It could be something like a magnet. And you could put some kind of a magnetic field on it, and figure out what the magnetization is. And this list of mechanical properties goes on. But you know that that's not the end of the story. You kind of expect that there are additional things that have to do with thermal aspects that you haven't taken into account. And as of yet, you don't quite know what they are. And you have to gradually build upon those properties.
So you have a system. These are kind of the analogs of the coordinates and potentially velocities that you would have for any Newtonian particles. A way of describing your idealized system. And then you want to find rules by which these coordinates are coevolving, or doing things together. And so for that you rely on observations, and construct laws of thermodynamics.
All right so this is the general approach. And once you follow this, let's say, what's the first thing that you encounter? You encounter what is encoded to the zeroth law. What's the zeroth law? The zeroth law is the following statement, if two systems-- let's call them A and B-- are separately in equilibrium with C-- with a third system-- then they are in equilibrium with each other. This is sort of this statement that the property of equilibrium has the character of transitivity. So what that means, pictorially, is something like this.
Suppose I have my two boxes, A and B. And the third system that we're calling C. And we've established that A and B are separately in thermal equilibrium with C, which means that we have allowed exchange of heat to take place between A and C, between B and C, but currently, we assume nothing-- or we assume that B and C are not connected to each other.
The statement of the law is that if I were to replace this red with green, so that I have also exchange that is going on between A and B, then nothing happens. A and B are already in equilibrium, and the fact that you open the possibility of exchange of heat between them does not change things. And again, this is this consequence of transitivity.
And one of its kind of important implications ultimately is that we are allowed now based on this to add one more coordinate to this description that you have. That coordinate is the analog of temperature. So this transitivity really implies the existence of some kind of empirical temperature.
And you may say well, this is such an obvious thing, there should be transitivity. Well, I want to usually give people examples that transitivity is not a universal property, by as follows. Suppose that within this room, there is A who wants to go on a date with C, and B who wants to go on a date with C. I'm pretty sure that it's not going to be the property that A wants to go through the date with B. It's 20%. All right.
So let's see how we can ensure this. So we said that if some system has reached equilibrium, it has some set of coordinates. Let's call them A1, A2, et cetera. There's some number of them. We don't know. Similarly, here, we have C1, C2, et cetera. And for B, we have B1, B2.
Now what does it mean that I have equilibrium of A and B? The implication is that if I have a system by itself, I have a bunch of possible coordinates. I can be anywhere in this coordinate space. And if I separately have system C, I have another bunch of coordinates.
And I can be anywhere in this coordinate space. But if I force A and B to come together and exchange heat and reaches equilibrium, that is a constraint, which means that this set of coordinates of the two cannot be independently varied. There has to be some functional relationship between them, which we can, for example, cast into this form.
So equilibrium, one constraint, one kind of mathematical relation. Similarly, equilibrium of-- this was A and C, B and C-- would give us some other function BC of coordinates of B and coordinates of C equal to 0. OK?
So there is one thing that I can do. I can take this expression and recast it, and write it as let's say, the first coordinate that describes C is some other function, which I will call big F AC of coordinates of A. And all coordinates of C, except the first one that I have removed. Yes?
AUDIENCE: When you put that function F AC or all the coordinates of A, and all the coordinates of C being zero, is that [? polynomy ?] always going to be true for the first law, or do you just give an example?
PROFESSOR: It will be always true that I have some set of coordinates for 1, some set of coordinates with 2, and equilibrium for the two sets of coordinates is going to be expressible in terms of some function that could be arbitrarily complicated. It may be that I can't even write this, but I have to graph it, or some kind of place in the coordinates space.
So what I mean is the following, you can imagine some higher dimensional space that is spanned by As and the Cs. And each point, where the things are in equilibrium, will be some-- you can put a cross in this coordinate space. And you can span the various places where equilibration takes place. And you will have a surface in this space. And that surface potential you can describe mathematically like this. OK?
And similarly, I can do the same thing. And pick out coordinate C1 here, and write it as F BC of A1, A2, and C2, C3-- sorry, this is B1, B2. Actually, this brings me, this question, to maybe one point that I should make. That sometimes during this course, I will do things, and maybe I will even say that I do things that are physically rigorous, but maybe not so mathematically.
And one example of this is precisely this statement. That is, if you give a mathematician and there is a function like this. And then you pick one, C1, and you write it as a function of all the others, they say, oh, how do know that that's even possible, that this exists? And generally, it isn't. A simple example would be A squared plus C squared equals to zero, then C's multiple valued.
So the reason this is physically correct is because if we set up this situation, and really these very physical quantities, I know that I dialed my C1 to this number here, and all of the other things adjusted. So this is kind of physically OK, although mathematically, you would have to potentially do a lot of [INAUDIBLE] to arrive at this stage. OK.
So now if I have to put all of those things together, the fact that I have equilibrium of A and B, plus equilibrium of B and C, then implies that there is eliminating C1 between these two, some function that depends on the coordinates of A and coordinates of C, starting from the second one, equal to some other function. And these functions could be very different, coordinates of B and coordinates of C starting from the second one. Yes?
AUDIENCE: Do you need A and C on the left there?
PROFESSOR: A and C, and B and C. Thank you. OK?
So this, everything that we have worked out here, really concerns putting the first part of this equation in mathematical form. But this statement-- in mathematical form-- but given the first part of this statement, that is, the second part of the statement, that is that I know that if I remove that red and make it green, so that heat can exchange, for the same values of A and B, A and B are in equilibrium, so I know that there is a functional form.
So this is equilibrium of A and B, implies that there is this function that we were looking at before, that relates coordinates of A and B that constrains the equilibrium that should exist between A and B. OK.
So the first part of the statement of the zeroth law, and the second part of the statement of the zeroth law can be written mathematically in these two forms. And the nice part about the first part is that it says that the equilibrium constraint that I have between A's and B's can mathematically be kind of spread out into some function on the left that only depends on the coordinates of A, and some function on the right that only depends on coordinates of B.
So that's important, because it says that ultimately the equilibration between two objects can be cast mathematically as having some kind of a function-- and we don't know anything about the form of that function-- that only depends on the coordinates of A. And equilibration implies that there exists some other function that only depends on the coordinates of the other one. And in equilibrium, those two functional forms have to be the same.
Now, there's two ways of getting to this statement from the two things that I have within up there. One of them is to choose some particular reference system for C. Let's say your C is seawater at some particular set of conditions. And so then, these are really constants that are appropriate to see water. And then you have chosen a function that depends on variables of A, of course of some function of B. Or you can say, well, I can replace this seawater by something else.
And irrespective of what I choose, A and B, there by our definition in equilibrium with each other, no matter what I did with C. Or some various range of C things that I can do, maintaining this equilibrium between A and B. So in that perspective, the C variables are dummy coordinates. So you should be able to cancel them out from the two sides of the equation, and get some kind of a form like this.
Either way, what that really means is that if I list all of the coordinates of, say, A, and I put it, say, in equilibrium with a bath that is at some particular temperature, the coordinates of A and B are constrained to lie in some particular surface that would correspond to that particular theta.
And if I have another system for B, the isotherm could have completely different for that data. But any time A and B are in equilibrium, they would be lying on the isotherm that would correspond to the same fate.
Now, again, a mechanical version of this, that is certainly hopefully demystifies any mystification that may remain, is to think a scale. You have something A on this scale. And you have something C on this scale. And the scale is balanced. You replace A with B, and B and C are in balance, then you know that A and B are in balance with each other.
And that implies that, indeed, there is a property of the objects that you put on the balance. You can either think of it as mass, or more appropriately, the gravitational force that they experience, that they need. The thing is balanced. The forces are equal. So it's essentially the same thing.
Now having established this, then you want to go and figure out what the formula is that relates the property that needs to be balanced, which is maybe the mass or the gravitational force in terms of density, volume, et cetera. So that's what you would like to do here. We would like to be able to relate this temperature function to all the other coordinates of the system, as we're going to. Any questions? Yes?
AUDIENCE: So how did [INAUDIBLE] that there is the isotherm? Is it coming from the second conclusion?
PROFESSOR: OK. So what we have said is that when two objects are in equilibrium, this function is the same between. So let's say that we pick some kind of a vat-- like, it could be a lake. And we know that the lake is so big that if you put something in equilibrium with that, it's not extracting too much heat or whatever from the lake can change its temperature function. So we put our system in equilibrium with the lake, which is at some particular fixed value of theta. And we don't know what theta is, but it's a constant.
So if I were to fiddle around with the system that I put in the lake-- I change its volume, I change its length, I do something-- and it stays in equilibrium with the length, there is some function of the coordinates of that system that is equal to theta. So again, in general, I can make a diagram that has various coordinates of the system, A1, A2, A3.
And for every combination that is that this theta, I will put a point here. And in principle, I can vary these coordinates. And this amounts to one constraint in however many dimensional space I have. So if we span some kind of a surface-- so if you're in three dimension, there would be a two dimensional surface. If you're in two dimension, there would be a line that would correspond to this constraint.
Presumably, if I change the lake with something else, so that theta changes, I will be prescribing some other curve and some other surface in this. Now these are surfaces in coordinate space of A. In order to be in equilibrium with an entity at the fixed value of theta, they prescribe some particular surface in the entire coordinate space and they're called isotherms. OK?
Actually, let's state that a little bit further, because you would like to give a number to temperature, so many degrees Celsius, or Fahrenheit, or whatever. So how do you do that? And one way to do that is to use what is called the ideal gas temperature space-- the ideal gas scale.
So you need some property at this stage in order to construct a temperature scale. And it turns out that a gas is a system that we keep coming back to again and again. So as I go through the various laws of thermodynamics, I will mention something special that happens to this law for the case of this ideal gas. And actually, right now, define also what I mean by the ideal gas.
So we said that a gas, in general, you can define through coordinates P and V. So if I put this gas in a piston, and I submerge this piston, let's say in a lake, so that it is always at whatever temperature this lake is. Then I can change the volume of this, and measure what the pressure is, or change the pressure, and figure out what the volume is. And I find out that there is a surface in this space where this curve that corresponds to being equilibrium with this [? leaves-- ?] this is the measure of the isotherm.
Now the ideal gas law is that when I go to the limit there, V goes to infinity, or P goes to zero. So that the whole thing becomes very dilute. No matter what gas you put here-- whether it's argon, oxygen, krypton, whatever-- you find that in this limit, this shape of this curve is special in the sense that if you were to increase the volume by a factor of two, the pressure will go down by a factor of two, such that the product PV is a constant.
And again, this is only true in the limit where either V goes to infinity or P goes to 0. And this is the property that all gases have. So you say OK, I will use that. Maybe I will define what the value of this product is to be the temperature. So if I were to replace this bath with a bath that was hotter than this product for the same amount of gas, for the same wire, would be different. And I would get a different constant.
You maybe still want a number. So what you say is that the temperature of the system in degrees Kelvin is 273 times 16. The limit of PV, as V goes to infinity of your system, divided by the limit of the same thing at the triple point of water [? iced. ?]
So what does that mean? So you have this thing by which you want to measure temperature. You put it in contact with the system-- could be a bath of water, could be anything, yes? You go through this exercise and you calculate what this product is. So you have this product. Then what you do is you take your system, and you put it in a case that there are icebergs, and there's water, and there will be some steam that will naturally evaporate. So you calculate the same product of PV in this system that is the triple point of ice water, et cetera.
So you've measured one product appropriate to your system, one product appropriate to this reference point that people have set, and then the ratio of those things is going to give you the temperature of the system that you want to measure. This is clearly a very convoluted way of doing things, but it's a kind of rigorous definition of what the ideal gas temperature scale is.
And it depends on this particular property of the diluted gases that the production of PV is a constant. And again, this number is set by definition to be the temperature of the triple point of the ice water gas. OK. Other questions?
All right. So this is now time to go through the first law. Now I'll write again this statement, and then we'll start to discuss what it really means. So if the state of an otherwise adiabatically isolated system is changed by work, the amount of work is only function of initial and final points.
OK. So let's parse what that means and think about some particular example.
So let's imagine that we have isolated some system. So that it's not completely boring, let's imagine that maybe it's a gas. So it has P and V as some set of coordinates. Let's say that we put some kind of a spring or wire in it, so we can pull on it. And we can ask how much we pulled, and what is the length of this system.
Maybe we even put a magnet in it, so we have magnetization that we can measure if we were to pass some kind of a current and exert some kind of magnetic field. So there's a whole bunch of coordinates that I'm completely familiar with from doing my mechanics courses and electromagnetic courses. So I know various ways to do mechanical work on this system.
So the system is otherwise isolated, because I don't really know how to handle this concept of heat yet, but I certainly have no problems with mechanical work. And so what I do is, I imagine that it is initially isolated. What I do is therefore, I have some-- in this case, six dimensional coordinate space. I'm will only draw two out of the six.
And I start some initial point, let's call it I. And then I start doing various types of things to this. I could, for example, first pull on this, so that the length changes, changes the current, put pressure so that the volume changes, et cetera. At the end of this story, I'm at some other point that I will call F.
Now I could have performed this change from the initial to the final state through one set of changes taking place one after the other. But maybe I will change that, and I will perform things in a different way. So there's path number 1. Then there's path number 2. And there's huge number of different paths that I can, in principle, take in order to change between the initial and final points by playing around with the mechanical coordinates that describe the system.
I always ensure that, initially, I was in equilibrium, so I could know exactly what the value of these parameters are. And finally, I wait until I have reached equilibrium. So again, I know what this things are.
And I know what mechanical work is. And I can calculate along each one of these paths, the net amount of work. The work is delivered in different ways-- through the magnetic field, through the pulling of the spring, to the hydrostatic pressure, et cetera-- but ultimately, when I add up all of the increments of the work, I will find that all of them will give you the same delta W, irrespective of the path. OK.
Now this reminds me of the following, that if I'm rolling a ball on top of a hill. And there is no friction. The amount of work that I do in order to take it from here to here, irrespective of the path that I take on the hill, it really is a function of the difference in potential energy between the final and initial points. So it's the same type of thing.
Rather than moving these coordinates on a hill, I am moving them in this set of parameters that thermodynamically describe the system, but I see the same thing that I would see in the absence of friction for rolling a ball of the hill. And immediately, I would deduce here that there is this potential energy, and the amount of work that I have to do to roll this off the hill is the difference between the potential energy between the two points.
So here, similarly, I would say that this delta W-- I define it to be the difference between some function that is called the internal energy-- that depends on the final set of coordinates. And there's a whole bunch of them. Think of them in some pictorial context. Minus what you have initially.
So in the same sense that the zeroth law allowed me to construct some function of coordinates that was relevant to thermal equilibrium, the first law allows me to define another function of coordinates, which is this internal energy. Of course, the internal energy is the remnant of the energy that we know in mechanical systems to be conserved quantity. And this is the statement of fact. So far, nothing surprising.
Now the real content of the first law is when we violate this condition. So essentially, what we do is we replace the adiabatic walls that were surrounding our very same system with walls that allow the exchange of heat. And I do exactly the same set of changes. So maybe in one case I stretch this, and then I change the volume, et cetera.
I do exactly the same set of changes that I did in this case, I try to repeat them in the presence of diathermic walls, go from the same initial state to the same final state. So the initial state is the same. The final state, I postulate to be the same. And what is observed is that, in this case, the diathermic walls-- which allow heat exchange-- that the amount of work that you have to do is not equal to the change in internal energy. OK?
Now you really believe that energy is a good quantity. And so at this stage, you make a postulate, if you like, it's the part of the corollary of the first law that allows you to define exactly what heat is. So you're gradually defining all of the things that were missing in the original formulation. You define this heat input to the system to be the difference in energy that you expected, minus the amount of work that you did in the presence of these walls. OK? Yes?
AUDIENCE: If we need the first law to the point of heat--
PROFESSOR: Yes.
AUDIENCE: --how did we define [? adiabatic ?] used in--
PROFESSOR: Right.
AUDIENCE: --first law and the zeroth law?
PROFESSOR: As I said, it's an idealization. So it's, in the same sense that you can say well, how would you define Newtonian mechanics that force is proportional to mass times acceleration, what is the experimental evidence for that? You can only do that really when you go to vacuum. So what you can do is you can gradually immerse your particle into more and more dilute systems, and see what is the limiting behavior in that sense.
You can try to do something similar here. You can imagine that you put your system in some kind of a glass, double glass, container, and you gradually pump out all of the gas this is between the two of them. So that, ultimately, you arrive at vacuum. You also have to mirror things, so there is no radiation exchange, et cetera. So gradually, you can try to experimentally reach that idealization and see what happens.
But essentially, it is certainly correct that any statement that I make about adiabatic walls is an isolation. But it's an isolation in the same sense that when you think about the point particle in Newton's laws. OK?
Let's go a little bit further with this. So we have that in differential form, if I go from one point in coordinate space that describes the system in equilibrium, where energy's defined to a nearby point, I can calculate what the value of the change in this energy function is. And I can say that there is a quantity, dE, that depends on a whole bunch of coordinates that define the system.
And what the first law says is that if you try to operationally make this change from one point to another point, you have to supply work through the system, or you have to supply heat through the system. And we write them in this form.
And what this D and d bar-- and we will encounter this many times in future also and define them better-- is that E is a function of state. It depends on where you are in this parameter space. So in the same sense that maybe you have a function of x and y, could be like x squared plus 3x y, I can define what dE is in terms of the x and y. So that's where I have this quantity.
But dW and dQ depend on precisely how this system was made to go from here to here. And you can sort of go between how much contribution to dE comes from here or from there, by certainly say, changing the properties of the walls from being adiabatic to being diathermal, et cetera. So these quantities really, as opposed to this quantity that depends on stage, these quantities depend on path, the conditions by which you implement a particular change.
Now there is a desire, and it's very important for us to actually construct what this function is. You want to, sort of, know what the energy function for, let's say, a mechanical system is. In a potential, you want to know what the form of E is and then you can do a lot of things with it. So how do we construct that for our thermodynamics system?
Again, we can idealize things and go to processes that are so-called, quasi-static. Which effectively means slow, or slow enough, to maintain equilibrium. And the general idea is, suppose I wanted to calculate what the potential energy of a spring is, or what the potential energy of a particle rolling on a hill is, well, one way that I could do that is I could, let's say, pull on this sufficiently slowly, so that as I'm pulling this by a certain amount, the spring does not start to vibrate. And so the force that I'm exerting externally, is really the force that is internal to the spring.
If I really push it to rapidly, the spring will start to oscillate. There's really no relation now between the external force that, let's say, is in uniform value and the internal force that is oscillating. I don't want to do that because when that happens I don't know where I am in this coordinate space. I want to do things sufficiently slowly so that I go from here to here here-- every time I am on this plane that defines my properties in equilibrium.
If I do that, then I can calculate the amount of work, and for the case of the spring, it would be the force times extension. And so we generalize that, and we say that if I have multiple ways of doing work on the system, there is the analog of the change in length of the spring-- that is the displacement of the spring, if you like-- multiplied by some generalized force that is conjugate to that.
And indeed, mechanically, you would define the conjugate variables by differentiation. So if you, for example, know the potential energy of the spring as a function of its length, you take a derivative and you know what the force is. So this is essentially writing that relationship for the case of the spring, generalize to multiple coordinates. And we can make a table of what these displacements are and the corresponding coordinates for the types of systems that we are likely to encounter.
So what is x? What is j? And the first thing that we mentioned was the case of the wire. And for the wire we can-- the displacement of the length is important, and the conjugate variable is the force with which you are pulling on this. In the first problem set, you will deal-- in the first test preparation, you will deal with the case of film. And for the film, what you do is you change the area, or if you have a balloon, you can blow on the balloon, and the surface area of the balloon changes. And there's a corresponding conjugate variable to that which is called the surface tension.
This is essentially the same thing, going from one dimension to two dimension. And if I were to go one dimension higher, in the case of the gas, I have the volume of the box. And I went through this just for the notation that the hydrostatic pressure of the work is defined to be minus p w-- minus PBV. Sorry. pW is minus PVV, as opposed to, say, the force of the spring, that is FDL. It's just, again, matter of definition and how we define the sign of the pressure.
And for the case of the magnet that we also briefly mentioned, we have here MDB. The one thing that you can see is the trend that all of these quantities, if you make the size of your system twice as big, these quantities will get proportionately bigger. So they're called extensive. Whereas the force and all the other quantities are intensive.
So what we've established is that if we focus only on these types of transformations, that don't include heat, we can relate the change in energy directly to dW. And the dW, we can write as the sum over i Ji dxi.
Now in order to really get dE, in general, I have to add to this dQ. And so really the question that we have is, is there some kind of analog of dW that we can write down for dQ? And if you think about it, if you have two springs that are in equilibrium, so that the thing does not go one way or the other, the force exerted from one is the same as the force exerted on the other. So in equilibrium, mechanical equilibrium forces, pressures, et cetera, are generally the same.
And we've established that when you are in thermal equilibrium, temperatures are the same. So we have a very good guess that we should really have temperature appearing here. And then the question that we'll sort of build on is what is the analog of the quantity that we have to put here.
Let me finish by telling you one other story that is related to the ideal gas. I said that for every one of these laws, we can kind of come up with a peculiar feature that is unique to the case of the ideal gas. And there is a property related to its energy that we will shortly explore. But let me first say what the consequence of these kinds of relations for measurable quantities that we can already try to deduce are.
So one thing that you can relate to heat, and properties of particular material, is the heat capacity. So what you can do is you can take your material and put some amount of heat into it. And ask, what is the corresponding change in temperature? That would be the heat capacity.
Now this bar that we have over here, tells me that this quantity-- which we will denote on C-- will depend on the path through which the heat is added to the system. Because we've established that, depending on how you add heat to the system, the change that you have in the coordinate space is going to be distinct potentially. Actually, we establish the other way that for a given change in the coordinate system, the amount of the Q depends on path, so they're kind of equivalent statements.
So if I'm dealing with a gas, I can add the heat to it, at least among many other possibilities, in two distinct ways. I can do this at constant volume or at constant pressure. So if I think about the coordinate space of the gas, which is PV, and I start from some particular point, I can either go along a path like this, or I can go along the path like this, depending on which one of these quantities I keep fixed. So then in one case what I need is the change in Q at constant v, dT. In the other case, this change in Q, a constant P, dT.
Now a consequence of the first law is that I know that dQ's are related to dE minus dW. And dW for the gas is minus PdV. So I can write it in this fashion. Divided by dT, and in one case, done at constant V, and in the other case, done at constant p.
The distinction between these two paths immediately becomes clear, because along the paths where the volume is kept constant, there is no mechanical work that is done along the this path, yes? And so this contribution is 0. And you have the result that this is going to be related to the change in energy with temperature at constant V, whereas here you have the change in energy, in temperature at constant P, plus P dV by dT at constant P.
So there is some additional manipulations of derivatives that is involved, but rather than looking at that in the general case, I will follow the consequence of that for a particular example, which is ideal gas expansion.
So as this is an observation called Joule's experiment. I take a gas that is adiabatically isolated from its environment. I connect it, also maintaining adiabatic isolation to another chamber. And initially all of the gas is here, and this chamber is empty. And then I remove this, and the gas goes and occupies both chambers. Observation, there is some temperature initially in this system that I can measure. Finally, I can measure the temperature of this system. And I find that the two temperatures are the same.
In this example, since the whole thing was adiabatically isolated, that the Q is 0. There is no mechanical work that done on the system, so delta W is also 0. And since delta is the same, I conclude that delta E is the same for this two cases.
Now, in principle, E is a function of pressure and volume. And pressure and volume certainly changed very much by going from that place to another place. OK, so let's follow that. So E we said is a function of pressure and volume. Now since I know that for the ideal gas, the product of pressure and volume is temperature, I can certainly exchange one of these variables for temperature. So I can write, let's say, pressure to be proportional to temperature over volume. And then rewrite this as a function of temperature and volume.
Now I know that in this process the volume changed, but the temperature did not change. And therefore, the internal energy that did not change can only be a function of temperature. So this Joule expansion experiment immediately tells me that while internal energy, in principle, is a function of P and V, it is really a function of the product of P and V, because the product of P and V, we established to be proportional to temperature. OK?
So now if I go and look at these expressions, I can see that these, dE by dT, and irrespective of V and P is the same thing, because E only depends on temperature. And since V is-- we know that PV is proportional to temperature, a constant P, dV by dT is the same as V over T. And so if I look at the difference between those two expressions, what I find is that this part cancels. This part gives me the value of this product, PV over T, which we said is a constant.
And it is certainly depends on the amount of gas that you have. And so you can pick a particular amount of gas. You can experimentally verify this phenomenon, that the difference of heat capacity along these two paths is a constant. That constant is the same for the same amount of gas for different types of argon, krypton, et cetera.
And since it is proportional, eventually to the amount of matter, we will ultimately see that it can be set to be the number of particles making up the gas in some constant of proportionality that we will identify later in statistical physics is the Boltzmann parameter, which is 1.43 times 10 to the minus 23 or whatever it is.
All of this depends partly on the definition that you made of temperature. What we will do next time is to review all of this, because I've went through them a little bit more rapidly, and try to identify what the conjugate variable is that we have to put for temperature, so that we can write the form of dE in a more symmetric fashion. Thank you.
Free Downloads
Video
- iTunes U (MP4 - 192MB)
- Internet Archive (MP4 - 192MB)
Subtitle
- English - US (SRT)