Flash and JavaScript are required for this feature.
Download the track from iTunes U or the Internet Archive.
Lecturer: Prof. Hazel Sive
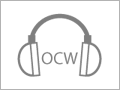
27: Nervous System 3
Related Resources
Biology terms (PDF)
All right. So let me move onto the last lecture in this module. Not in the module. In the last lecture in the nervous system part of the systems module. And I want to start by talking briefly about learning and memory, very briefly about learning and memory.
We talked last time about setting up synapses, we talked about making connections between the wires, and we talked about how changes in membrane potential could either determine whether a synapse fired or whether it did not.
However, there's more to it than that. There is significant data that indicates that the more often you use a synapse the strong that synapse will become. What do I mean by stronger?
Well, I'm actually not sure what I mean by stronger, except in a couple of cases that I'll tell you about.
So the term I want to, so synaptic connections, this is going to be long. Synaptic connections strengthen with use.
And the phenomenon I want to tell you about is long-term potentiation or long-term depression.
And this is a phenomenon that was discovered in neurons that were studied in isolation.
So it's very difficult, it's impossible to study neurons within the brain of any complex animal, especially mammals. And so what's done is to use so-called slice cultures.
Where you take a slice out of the brain, a very thin slice that's just got a few layers of cells, and you look and see what these neurons do in some kind of plastic tissue culture dish with media.
And they actually do quite a bit. The neurons fire and they make synapses. And a lot has been learned with them, with these kind of slice cultures. So here's the deal.
If one has a cell, if you look at this picture here, if one has a cell and one stimulates the synapse you see that there is a response to the first stimulus.
And you're actually measuring change in potential in the cell. Let's not worry about how the experiment was done because we're not going to get through the material I want to cover today. You can come see me in office hours and I'll explain to you.
What I want to point out is that after a first stimulus there is a change in the potential of the responding cell.
If you continue to give that stimulus, you get a much stronger response later on.
So the strength of the response, the change in membrane potential in a responding cell increases with stimulation of that presynaptic cell, of that synapse. And that is what long-term potentiation is.
It increases the chances that the postsynaptic cell will fire.
And it is believed that long-term potentiation has got a profound role in memory and in learning. So how does that actually work? Well, it's not clear in most cases, but this is an example from your book that is the shining example of how learning and memory can work at the molecular level.
And it has to do with glutamate. You do not have this on today's handout. It was on your last lecture's in-class handout.
But you can look up here and then go back to your last lecture's handout if you don't have it with you.
It has to do with glutamate. So glutamate is the major excitatory neurotransmitter in the central nervous system. And glutamate activates two different ionotropic receptors. One of them is called the AMPA or the non-NMDA receptor and the other is called the NMDA receptor.
With low level of stimulation or with single stimulation of a presynaptic neuron the AMPA receptors are activated. And these are sodium channels, so these are ionotropic channels. And glutamate is released, binds to the AMPA ion channel, changes its confirmation, and you get an influx of sodium, and therefore get closer to threshold potential.
However, on the same synapse, like right next door are these other receptors called the NMDA receptors. And they are not used if the presynaptic cell is stimulated at a low level or infrequently.
But if you keep stimulating that presynaptic cell, eventually these NMDA receptors will open.
And they will open and they will allow an influx of both calcium and sodium. And what happens is that you get a much stronger change in potential because you've got now two sets of receptors open.
And this postsynaptic cell is much more likely to fire and is actually much more stable in the synapses that it has made.
So this NMDA channel only open after repeated stimulation. So that's an example of what repeated stimulation can do to changing channels. And there is some molecular data, although it's really not complete, as to why you switch this channel usage.
One thing, however, that's very interesting about long-term potentiation, and indeed about learning and memory anyway, is that you need --
-- protein synthesis for learning especially. For classical learning, the kind of learning that you do before a test, the kind of learning that a mouse does to find its way through a maze repeatedly requires protein synthesis.
And this is very interesting because what I've been telling you about in this module is stuff that happens in the neuron that is actually independent of transcription or protein synthesis.
It involves changes in gene activity.
It can involve changes in gene regulation, as I'll talk about in a moment, but it does not actually require any transcription or protein synthesis per se. Learning and memory of the classical kind that we think about does.
And it's one of the reasons that you need to get a few hours sleep before you take a test.
It's one of the reasons if you pull an all-nighter, and there are many, you do not do that well the next day because you need this round of protein synthesis that does stuff that probably synthesizes new receptors that allows the synaptic connections you've made through your learning to be strengthened.
And that's still a mystery, really, as to what the protein synthesis is required for. But if you're interested, this work from [Ed Plezia?] that I mentioned at the beginning of class has shed some really fascinating light on it.
So come see me if you want to. All right.
So I want to move on now to circuitry. All right. I'll show you this slide because it's up there. This is a piece of data that I pulled down that's kind of interesting.
This looks at the AMPA NMDA ratio, receptor ratio with cocaine. So in a control there are about the same number of AMPA and NMDA receptors on a particular synapse. It's very interesting in some studies with cocaine use, you see the amount of the AMPA receptor.
That is the receptor that is the short-term receptor increases relative to the NMDA ratio, an NMDA receptor level, indicating that with cocaine you might not be as good at learning as without cocaine.
It is not clear. There are a lot of studies like this and the interpretations of them are complicated, but this is a solid piece of data that you do change the ratio of the non-NMDA and the NMDA receptors.
All right.
So let's move on. So let's talk about circuitry. We've talked about the wires, the passage of current along the wires, we've talked about the connections between the wires, and now I want to talk about the circuits.
And I want to start by asking you a question. In thinking about your brain and how much of your brain you used in circuitry, how many of you were taught that you use one-tenth of your brain?
Raise your hands.
I want to see what you've been taught at schools. It's no reflection on your teachers. It's OK. Thank you. It's a substantial portion. I feel that it is my duty as your professor to debunk that myth once and for all forever.
It's not true. And you might want to actually email your high school teachers and tell them it's not true. OK. And you perhaps even learned about the boy who used, you know, one-fifth of his brain and was an unbelievable genius.
There is, none of this, I was taught that as well, and it is absolutely untrue.
And the data for it is very solid. For example, any neurosurgeon who goes into your brain knows that no matter what part of the brain he or she goes through to remove your brain tumor or do whatever it is, is going to damage some aspect of your brain function.
Anyone who has any kind of stroke, any kind of brain hemorrhage, any kind of leakage of blood into the brain is going to have some kind of deficit. It might be a small one or not.
But there is ample evidence that you use all your brain.
And, indeed, why would one go to the trouble of making a brain that is so large if one was not using it? So there we go. Let me talk a little bit about circuitry. To indicate the complexity of circuitry in your brain let me point out what parts of your brain it takes to put together language.
If you're looking at words, it takes this area right at the back of your brain, the base of your brain.
Listening to words takes a different region. Speaking words takes a different region. Generating words take different regions.
And these are very, these are PET scans. We can talk about what they are. They measure the energy usage in your brain. And these are very, very large parts of your brain. OK? These are millions, close to billions of neurons involved in each part of working with language.
OK? And you can go to your brain and you can actually, people have mapped these quite finely.
So the circuitry in your brain is enormous, and the effort to both set up and maintain the circuitry is enormous.
Here is another interesting piece of information. About 20% to 25% of all the energy that you use is used by your brain, which is one of the reasons you probably need to sleep. It's not clear why we need to sleep.
But it is a tremendous, there takes a tremendous amount of effort to get the wiring set up and to keep the wiring set up and to keep the circuitry running.
Here's another example of circuitry that I'm going to come back to.
It's called a retinotopic map. And I'm going to start talking about it by addressing the question of how the circuitry in your nervous system is set up in the first place. And I'm going to use this as an example throughout the rest of the class.
So when people realized way back when that there were circuits set up in the nervous system, the question was how did these circuits get set up? How did neurons know which connections to make? And there were two competing theories.
One I'm going to call the random/survival theory.
They've got lots of names in the literature, but I call it the random/survival theory.
And the notion here was that neurons grow all over the place, and if they make connections that work, if they're productive connections, those connections will be maintained, the neurons will survive, and there you go.
You have your circuit. The other theory is the guidance theory, that there's actually something telling the neurons where to go.
And I'll show you a piece of data that suggested that this guidance theory was really correct.
But today, in fact, both of these theories seem to be correct when put together in a way I'll try to build up for you. So your eye, your eye and your visual system are very complex. Your eye starts off sort of like a camera, but very rapidly that analogy breaks down.
And light falls onto your retina and the photoreceptors in your retina, and from your retina a series of axons are bundled together to make the optic nerve, which goes to your brain.
And, in fact, I should point out, I'm not going to talk more about this, but the image that your retina sees we know quite clearly, a Nobel Prize was given for it, is not the image that you see when you look at me or you look around the room.
It's a series of shadows and bright points and so on.
It's really not a direct camera image as the camera does it for us. But that is no matter now. What is important is that some set of stimuli go from your retina through the optic nerve back to your brain.
And they go to two places in your brain. So here are some diagrams. Let's look at the very left most one. This isn't on your handout but look at it up here.
I've got an equivalent one later on. So in your eye, from your eye the axons from the eye are sent back, project back to a region in your brain called the tectum.
It's got a lot of different names, lateral geniculate nucleus or the thalamus or the tectum. OK. We'll call it the, I think I'm calling it the tectum in this lecture. Let me see. Let me make sure I've got it correct.
Yeah, I'm going to call it the tectum.
OK? So we'll talk about the tectum. And from the tectum connect synapses are made, another set of neurons go back to another region of your brain called the visual cortex. And it's the summation of all these different connections that somehow allows you to interpret what you see and see it.
It's extremely complicated. What I want to concentrate on are the connections between the retina and the tectum.
And what's interesting in ourselves and any animals that see binocularly, that can see 3-dimensionally, is that the reason you can see in 3D is that some of the neurons that come from your right eye go to the right side of the brain and some of them go to the left side of the brain and vise versa.
Some of the neurons on the left side of your brain go to the left side, yeah, and some of them go to the right side. And so if you look in some, just look at the diagram so you're with me.
If you look in some, you'll see that some of the neurons go to the same side and some cross over and go to the other side.
OK. So you can look at this in a different way. This very beautifully labeling experiment where you can actually label neurons in different parts of the retina, and you can follow where they go. The label is maintained in their axons.
And as the axons, well, I'll talk about in a moment.
As the axons send out their processes back into, oh, I've called it the thalamus here, back into the thalamus then you can see those same axons.
So let's look here. This is the left eye and the right eye. What? The left eye and the right eye. OK. And let's look here at the images that you're going to get here on the left side, the connections you're going to get on the left side of your brain that will give you binocular vision in your right visual field.
Here are a set of neurons.
They're called the nasal neurons because they're on the side of your retina that's next to your nose. And these red neurons are going to send their axons back into the left side of the brain. Here they are.
And the same time the neurons from the left eye, from the side of your eye nearest the outside called the temporal, are going to send their axons back to the left side or to a different side of this thalamus or this tectum.
OK? So what I want you to see is red can go to one side. Excuse me. The red here cross over when they go to that side and the green cross over and go to that side. So let me diagram this more clearly so that we can actually discuss this with the diagram in front of you.
This is number one on your handout. OK? So let's look at this and indicate it more clearly.
These N normally in setting up binocular vision, this circle here is meant to be one eye, and this back here is meant to be one side of the tectum.
Let me write on the board that in retinal tactile mapping the tectum can also be called the thalamus.
All right. So what's important here is that these N neurons go back to this region of the tectum called the C.
OK? And I see I have not wrote, I have not indicated tectum here or retina. I'll go back and do it. But you should write here, the circle here is the retina and this oval here is the tectum. And then we will be together.
So these N neurons from the retina send out axons that get to this C or caudal region of the tectum.
And these T neurons or temporal neurons from the retina send out axons that get to this R or rostral region of the tectum. So this is a long preamble to tell you two things.
Firstly, these connections are what you always see in every animal with either binocular or monocular vision.
Now, the way this is set up is actually quite confusing. And I want to, let's not worry about the details too much, but let's look at, as I see it I realize it's quite confusing. I took it from a very old paper, which I thought would be of interest, but as I see it here there's one point of confusion.
But what I want to point out is an experiment that was done a long time ago by a guy called Roger Sperry which addressed this question of whether axons were guided in a particular way or whether or not they randomly found their way.
And then by some kind of survival cue the connections in the nervous system were set up. So what Sperry did was to take this tectum and he rotated it 180 degrees. So the C is now 180 degrees reversed.
And if he did that he had to break the axons that connected the normal retina and the tectum.
OK? But what happened was that those axons grew back. This was done in frogs and they grew back. And there are two possible outcomes to this experiment. The first is that these nasal, don't you love it?
Thank you so much.
OK. The first is that these nasal neurons will grow back to this R region, which they would not normally do, but it's far away from them so maybe that's how they work. The second is that these nasal neurons will grow back to the C region, N to C in the same way that they would in the normal case, even though they're closer together.
And the outcome of the experiment was unequivocal and it was fantastic and it was one of the reasons he got a Nobel Prize, which was that you always get these nasal neurons growing back to the C region and these temporal neurons growing back to the R region.
All right? So this is a very long preamble for a very complex axon writing system, but the bottom line here that I want you to have is that these retinal neurons knew which part of the brain they had to project back to.
And that was very strong evidence that there was guidance of these neurons to the correct region of the brain.
All right. So let's move on to how this guidance takes place. And I'm going to come back at the end to talk about survival because that's also an important part of this. And the part of the cell that you need to know is the growth cone.
Now, some lectures ago I gave you a lecture about 3-dimensional structure.
And we talked in some detail about cell migration and how cells move in a mechanistic sense. What we're going to talk about now is very related to that, but instead of talking about a whole cell moving we're going to talk about just part of a cell moving.
We're going to talk about this growth cone. So what is the growth cone?
The growth cone is the axon tip. It has both sensory function because it's got various receptors of the type we've talked about, more in a moment, and it also has motor function because it has all those actin-based microfilaments that allow it to move in exactly the same way that we saw the whole cell moving.
But you remember, of course, that axons are long and they're far away from the cell body, so we're just talking about the axon growing now.
This is number two on your handout, and this is a diagram of the end of an axon in the growth cone. The axon's structure, the length of the axon is stabilized by microtubules. And subsequent to the ending of where the microtubules are, the cell sends out a series of processes that are called filopodia or lamellipodia.
I've given you the singular here.
Filopodia are thin. Lamellipodia are fatter. It doesn't matter. And it's in these filopodia and lamellipodia that there are microfilaments that, you should know, are comprised of actin polymers. And I've shown you also receptors on this growth cone that can bind two molecules in the extracellular matrix or can bind two molecules on another cell.
OK.
This is what a growth cone really looks like. This is a time-lapse of minutes. And you can see that this is a very dynamic structure. The cell is sending out processes and retracting them, sending them out and retracting them.
And the sense that one has when one watches axons sending out their processes is that they really are feeling around their environment and trying to decide where they should go.
I want to remind you this is a diagram from one of your previous lectures from Formation 4. The processes by which the axon is moving and sending out its processes are exactly the same as the processes that change during mesenchymal cell migration, but we're just talking about sending out part of the membrane and not the whole cell moving.
All right. So what is important in this process? Well, what seems to be important are several things. OK.
And one set of things that are important are called short-range guidance cues. Short-range refers to how far they can work.
Whether these are diffusible molecules or not. And I'll talk about long-range ones in a moment. The distinction between them is a little semantic.
But short-range guidance cues include things in the extracellular matrix and include things that are irrevocably stuck onto cells so that you can really only get interactions directly between two adjacent cells or between a cell and something that it is directly just opposed to.
And I want to talk about two classes of cues.
Oh, and them I'm going to indicate later on long-range guidance cues. So let me first talk about cell adhesion molecules.
We've talked about the extracellular matrix a bunch.
Laminin, in particular, is a very important molecule for guiding axons through their paths to where they are going.
This is a movie showing an axon. This ought to be a movie showing an axon trying to decide what substrate it wants to migrate on.
Here is an axon. There it's sending out its growth cone. And this axon has been put onto a dish that's got two different kinds of coatings. On the top half it's got laminin and on the bottom half it's got polylysine.
And if you look at this you can see that it's sending out processes that are touching the polylysine, but as it goes on it decides it's really not interested in interacting with the polylysine and it's interested in interacting with the laminin.
And that's where it lands up. Other things that you should be aware of that are important in axon guidance in terms of cell adhesion are the molecules cadherins that we talked about previously.
Now, some of these are attractive.
In other words, axons will like to grow on a particular substrate with particular cell adhesive, with particular cell adhesive or cell interactions.
So attractive cues will promote axon outgrowth.
And one can counter this with repulsive cues or repulsive signals which will inhibit axon outgrowth. And this is going to be true both for the cell adhesion molecules I'll talk to you about and for other molecules later on.
So let's move onto diffusible molecules or the longer range molecules.
OK.
And I want to give you, to illustrate this, an example of a very beautiful axon guidance phenomenon. And so we're on number three on your handout. And this is the example I want to discuss with you.
It has to do with two sets of neurons in the spinal cord and the brain that are called commissural neurons and trochlear neurons.
This diagram is a section, it's a cartoon of a section through a spinal cord.
Spinal cord transverse section. If you lay on the ground and someone cuts you in half, that way that is a transverse section. OK? So here's the hole in the middle of the spinal cord, this is the roof plate of the spinal cord nearest the skin, and something called the floor plate which is deepest in your body.
The commissural axons grow from this dorsal region down towards the floor plate.
In contrast, these trochlear axons that I've shown in blue here grow from the floor plate away towards the roof plate. And this data is shown very beautifully by staining these cells with appropriate markers here.
And I apologize if you're red-green color blind. I know this is an issue, but this is how the stains are actually done. These are the real colors of the stains. So up here in the top of the spinal cord, these red dots are the cell bodies of neurons.
And the green are the axons that are sending out their processes. And these are the commissural axons and they are growing down, down, down towards the floor plate. And actually eventually will cross to the other side of the embryo.
So how do these axons know where to go?
Well, one model, one model suggests that these axons are being told where to go by this little region at the bottom called the floor plate. And this was tested in the following way.
One can go into a developing spinal cord, and one can cut out different pieces of it. So you can cut out a piece of the dorsal spinal cord and you can stick it close to a piece of the floor plate or you can stick it close to a piece of the roof plate.
And then you can look and see what happens.
And what happens with time is that neurons will develop in these pieces of dorsal spinal cord. In the case of a set of tissues that's got the dorsal spinal cord and the floor plate, you can see very clearly that axons will grow out towards the floor plate.
However, when you have the roof plate there, the axons do not grow out from the spinal cord.
There's no outgrowth. And this type of experiment indicated that the floor plate was attracting these commissural axons to it, and they were growing out because of this attraction. And here's what a real experiment looks like.
Here's a piece of dorsal spinal cord, here's a piece of floor plate, and here in the control experiment there was dorsal spinal cord.
And this is just a chunk of tissue culture cells instead of the roof plate.
And what you see here are these streaks coming out from the dorsal spinal cord explants towards the floor plate. And these streaks are the axons growing out. So this is actually a very robust assay.
And so using this assay, some years ago Marc Tessier-Lavigne's lab tried to find what the floor plate was secreting to attract these commissural axons.
And it was a long, hard road that involved dissecting 35, 000 chick brains in order to do biochemistry to get the proteins that were doing this attraction of these commissural axons.
But they got something in the end. And what they got is a protein called netrin-1. Panel B is a section through the spinal cord, and you cannot see it very well, except for this black glob at the bottom.
But what this is the floor plate.
And it's been stained for netrin-1 RNA. And you can see this netrin-1 RNA is only in the floor plate region. And if you look at the protein, it's also in the floor plate region.
Although, it diffuses a little bit out of that region. Is netrin-1 really important for making these axons grow in the right place? Well, yes. Because if you take a mouse and knock out, you genetically mutate the netrin-1 gene, the commissural axons don't grow properly.
So here's a wild type mouse spinal cord, here are these axons growing down to the floor plate, and here is a mutant mouse that's lacking netrin-1. And these commissural axons grow but they never get to the floor plate. They don't really know where to go. Now, if you look back at handout three --
-- there was something else about this floor plate region in that these trochlear axons were growing away from the floor plate.
And so one could construct the ultimate hypothesis that the floor plate was actually repelling these trochlear axons from growing towards it. And it turns out that is exactly what's happened, and netrin-1 can act both as a chemo attractant and a chemo repellant.
And it does so, and this is number five on your handouts.
It does so by using two different receptors, one of which makes it attractive to neurons and the other which makes it repulsive. So here's netrin indicated as a diffusible molecule. It binds to a receptor called DCC which was initially implicated in colon cancer called deleted in colon cancer.
It's not clear if it really is still involved in cancer or not.
But here's netrin binding to its receptor. And subsequent there are a bunch of signal transduction that happens that changes whether or not these axons move to the floor plate and whether or not they, in fact, grow out at all.
In the case of repulsion, it turns out that there's another receptor called unc-5. And that makes a connection with the DCC receptor. There's an interaction between the unc-5 and the DCC receptor.
Netrin still binds to the DCC receptor, but the net result of this is a different signal transduction pathway activated such that you now get repulsion of these neurons by activation of this duet of receptors.
So for this diffusible process, I've given you the example of netrin that can act both as an attractant and a repellant.
And many, I have no idea how to spell repellant. Repellant, I think it's got an E.
OK. Repellant. And there are now several receptors known that are able to be, there are several guidance cues known that can either be attractants or repellants. What happens when a growth cone sees a repulsive signal? This movie will show you.
That is the growth cone collapsing. Let me show it to you again.
So you start off with a pretty robust growth cone, and it collapses when you put a little drop of some guidance cue that it doesn't like up in this corner of the slide.
And what happens is that that actin cytoskeleton that is there just collapses, depolymerizes, and that axon tries to get as far away from the repulsive cue as it can.
So we've talked about short-range and long-range guidance cues.
We've talked about attractive and repulsive guidance cues. We've talked about how one single guidance cue can be both an attractant and a repellant. And now I want to get back to this very old hypothesis that the way that you actually got synaptic connections and the way you've got circuitry set up was by some kind of random way that involved survival of neurons that made the appropriate connections.
In fact, that is true once you get neurons to where they're finally going.
So you're setting up neurons, they're going along their path set up by some guidance cues. And of course you should be asking the question, well, how do the guidance cues get there in the first place, right? OK? So that's a question you can go and sit and think about.
But in the end what happens is that you stabilize connections.
And you do it in two ways.
Both of which, or one of which seems to be activity dependent, or another of which is activity independent.
By activity dependent, I mean that the nerve has to fire.
There has to be synaptic activity. And that will maintain the connection. If there is not synaptic activity you will not. And there are some cases where that is not the case.
This is number six on your handout. When neurons grow out to the neuromuscular junction they form a synapse, or when the neurons grow out to the muscle they form a synapse with the muscle. The synapse is initially a rather weak one, and over time there are proteins that are activated.
Acetylcholine receptors, for example, that are activated that set up the synapse and stabilize it and maintain it.
So that is one example of stabilizing connections. Another very famous example is growth factors that are survival signals. One of the most famous is NGF which stands for nerve growth factor.
And this is a protein that is involved in making certain sets of neurons survive when they get to their targets.
There seems to be a limiting amount of nerve growth factor around in specific regions of the animal, and only those neurons that can capture enough of that nerve growth factor on their receptors will survive.
So there are neuronal survival signals that may or may not require a neuronal activity for their effect. OK.
So two final things. One is this retinotectal map. We talked about the outcome of the Sperry experiments and how you got the neurons going back to where they are.
What's the molecular basis for that? Well, you can show very beautifully that the neurons prefer to grow on a particular part of membrane that you give them. So, for example, the retinal neurons will choose to grow on the anterior rostral and not on the more posterior caudal neurons.
And you can show that the reason for that is due to a class of molecules called the ephrins.
Ephrins are secreted molecules, but they are short range. They work just on the cell next door. And you can show, I'm flashing this up, I know you can go and look at it later on, that wherever there is ephrin you do not see axonal outgrowth.
So ephrins are inhibitory to axonal outgrowth.
And I'm going to refer you to, I'm not going to go through it. I'm going to refer you to number eight on your handout. I'm not going to go through this. You can look at it. But I will just tell you that there are different combinations of ephrins and Eph receptors which guide retinal axons to the correct part of the tectum.
The last thing that I want to spend the last minute on is a particularly fascinating point. And that is the question of nerve regeneration, and nerve regeneration in the central nervous system. So we believe right now that the same, in fact, we know that the cues that tell axons where to go in the first place in an embryo are maintained in the adult nervous system.
And we also know that if you cut one of the nerves in your peripheral nervous system in your arm it will grow back fine.
But we also know if you sever your spinal cord it will not grow back. And there has been intense research to try to figure out what it is that is making the axons in your spinal cord not grow back to where they were, although, in fact they should.
Here's a diagram of an intact CNS axon. If it's severed of course you're going to get a fragment that's not connected to a cell body, but the other part of it is still connected to the cell body.
And what happens is that that dies, that cell dies.
It undergoes apoptosis and dies. And it's really not clear why it doesn't regenerate. One hypothesis is that there it is a poor growth environment in the extracellular matrix around the axon in the CNS.
Another class of proteins of great interest are the Nogos and related ligands which are believed to be inhibitory to axon regrowth. And there's a lot of interest in this kind of thing.
And I'll stop there.
Thanks.
Free Downloads
Audio
- iTunes U (MP3 - 21MB)
- Internet Archive (MP3 - 21MB)
Subtitle
- English - US (SRT)